Block copolymer synthesis using a commercially available nitroxide-mediated radical polymerization (NMP) initiator
Nam S. Lee, Karen L. Wooley
Departments of Chemistry and Chemical Engineering, Texas A&M University
Material Matters 2010, 5.1, 9.
Introduction
Controlled radical polymerization, which provides exquisite tuning of macromolecular size, structure, composition and architecture, with experimental convenience, has become one of the most indispensible tools for polymer chemists. Its emergence in the mid-1990s has greatly advanced the fields of nanoscience and nanotechnology, by providing ready access to complex polymers that serve as building blocks for functional nanostructures with predictable parameters such as the size, morphology, regioselective placement of functionalities, etc. This exceptional polymerization control is due to reversible termination events that mediate the radical concentration and reactivity. The living character of this type of polymerization provides an ability to produce polymers with controlled molecular weight and narrow molecular weight distribution, and, moreover, to chain extend with different monomers and obtain multi-block copolymers. Nitroxide-mediated radical polymerization (NMP) is one of these controlled radical polymerizations that also includes atom transfer radical polymerization (ATRP), and reversible addition-fragmentation chain transfer (RAFT) polymerization. NMP stands out due to its simplicity: the polymerization is thermally initiated in the absence of an external radical source or a metal catalyst. NMP involves a combination of radical initiator (Ι), monomer (M) and nitroxide radical (R), for trapping of intermediate radical species (Scheme 1). For instance, the thermally-promoted homolysis of benzoyl peroxide (179981) produces radicals that are capable of initiating the polymerization of styrene monomer. Propagation proceeds to produce polymer chains, while reversible termination events, involving reactions with nitroxide radicals to afford thermally labile alkoxyamines, mediate the availability of the reactive radical species and, thereby, provide control over the polymerization. It is important that the stable nitroxide radicals are capable of the reversible termination reactions, but do not initiate polymerizations.

Scheme 1.Overall mechanism for NMP, illustrated for styrene monomer (M) polymerization initiated by benzoyl peroxide initiator (Ι) and mediated by TEMPO nitroxide radicals (R). Also shown is the general structure for alkoxyamine-based unimolecular NMP initiators.
One of the most significant advances with NMP was the isolation of an alkoxyamine that could act as a unimolecular agent, providing both the reactive, initiating radical and the stable, mediating nitroxide radical. Initially, nitroxides were employed as additives to reversibly terminate polymer chains initiated by a separate radical source. By using TEMPO to trap a styrenyl radical initiated by benzoyl peroxide (Structure A of Scheme 1), Hawker demonstrated an ability to tune the molecular weight, define the end groups, and extend to block copolymers, while maintaining narrow molecular weight distributions. He later developed a universal initiator, which has received broad application in laboratories around the world. A key limitation to the use of this universal initiator remained the challenge of its synthesis. With it now being offered commercially, it is expected that NMP will experience a renewed vigor of investigation. With our interest in the construction of nanoscopic objects via the selfassembly of amphiphilic block copolymers in water, we have used the universal initiator (700703), in the presence of less than 5 equivalent percent of the corresponding nitroxide (added to assist with capping the propagating chain ends during polymerization), to prepare an amphiphilic diblock copolymer precursor, poly(tert-butyl acrylate)-b-poly(4-acetoxystyrene) with a controlled molecular weight and a narrow molecular weight distribution (Scheme 2).
Synthesis of Poly(t-butyl acrylate)140 (I)
1H NMR and 13C NMR spectra were recorded at 300 MHz and 75 MHz, respectively, as solutions with the solvent proton as a standard. To a flame-dried 50 mL Schlenk flask equipped with a magnetic stir bar and under N2 atmosphere, at room temperature, was added (124 mg, 0.381 mmol, 700703), 2,2,5-trimethyl-4-phenyl-3- azahexane-3-nitroxide (4.19 mg, 0.019 mmol, 710733), and tert-butyl acrylate (10.16 g, 79.6 mmol, 327182). The reaction flask was sealed and stirred for 10 min at rt. The reaction mixture was degassed through three cycles of freeze-pump-thaw. After the last cycle, the reaction mixture was recovered back to rt and stirred for 10 min before being immersed into a pre-heated oil bath at 125 °C to start the polymerization. After 36 h (kinetic data for conversion shown in Figure 1), 1H NMR analysis showed 50% monomer conversion had been reached (Figure 3). The polymerization was quenched by quick immersion of the reaction flask into liquid N2. The reaction mixture was dissolved in THF (401757) and precipitated into H2O/MeOH (v:v, 1:4) three times to afford PtBA as a white powder, (4.1 g, 80% yield based upon monomer conversion); Mn NMR = 19,130 g/mol, Mn GPC = 18,220 g/mol, PDI = 1.10. 1H NMR (CD2Cl2, ppm): δ 1.43 (br, 1290 H), 1.80 (br, 70 H), 2.21 (br, 160 H), 7.14-7.26 (m, 10 H). 13C NMR (CD2Cl2, ppm): δ 28.4, 36.5, 38.0, 42.5, 80.9, 174.4. The GPC data can be seen in Figure 2.

Figure 1.Percent conversion of monomers vs. time

Figure 2.Molecular weight distribution of I. Mn = 18,220 g/mol, PDI = 1.10

Figure 3.1H NMR spectrum of I
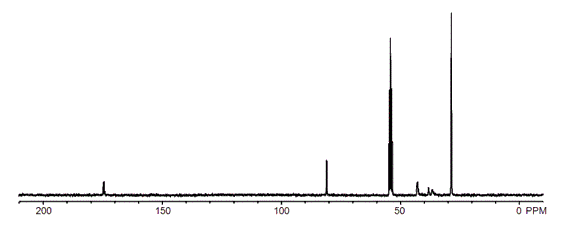
Figure 4.13C NMR spectrum of I
Synthesis of Poly(t-butyl acrylate)140-bpoly(acetoxystyrene)50 (II)
1H NMR and 13C NMR spectra were recorded at 300 MHz and 75 MHz, respectively, as solutions with the solvent proton as a standard. To a flame-dried 50 mL Schlenk flask equipped with a magnetic stir bar and under N2 atmosphere, at room temperature, was added I (124 mg, 0.381 mmol), 2,2,5-trimethyl-4-phenyl-3-azahexane-3-nitroxide (4.19 mg, 0.019 mmol), and 4-acetoxystyrene (10.16 g, 79.6 mmol, 380547). The reaction flask was sealed and stirred for 10 min at rt. The reaction mixture was degassed through three cycles of freezepump- thaw. After the last cycle, the reaction mixture was recovered back to rt and stirred for 10 min before being immersed into a preheated oil bath at 125 °C to start the polymerization. After 4 h (kinetic data for conversion shown in Figure 5), 1H NMR analysis showed 25% monomer conversion had been reached (Figure 7). The polymerization was quenched by quick immersion of the reaction flask into liquid N2. The reaction mixture was dissolved in THF and precipitated into H2O/MeOH (v:v, 1:4) three times to afford PtBA-b-PAS as a white powder, (4.62 g, 87% yield based upon monomer conversion); Mn NMR = 26,620 g/mol, Mn GPC = 26,330 g/mol, PDI = 1.12. 1H NMR (CD2Cl2, ppm): δ 1.43 (br, 1500 H), 1.80 (br, 100 H), 2.21 (br, 290 H), 6.36-6.82 (m, 190 H), 7.14-7.26 (m, 10 H). 13C NMR (CD2Cl2, ppm, Figure 8): δ 21.5, 28.4, 36.5, 38.0, 40.5, 42.6, 80.9, 121.8, 128.9, 143.0, 149.4, 169.7, 174.7. The GPC data can be seen in Figure 6.

Figure 5. Percent conversion of monomers vs. time

Figure 6. Molecular weight distribution

Figure 7. H NMR spectrum of II

Figure 8. C NMR spectrum of II
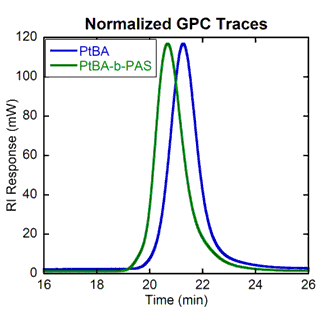
Figure 9.Normalized GPC traces showing molecular weight distributions of polymers I and II
Conclusions
We have demonstrated a facile preparation of an amphiphilic diblock copolymer precursor with a controlled molecular weight and a low PDI using the universal NMP initiator (700703). This required no special apparatus or technique, beyond those employed for standard radical polymerizations, but only synthesis of the corresponding nitroxide (710733). The final block copolymer was purified by precipitation to remove excess monomers, and was then deprotected. The morphology and size of the subsequent supramolecularly assembled nanostructures in water depend on the polymer block length and the ratio of the block lengths, each carefully manipulated through monomer conversions, the control over which arises from the universal NMP initiator. With the simplicity of this system, it is expected that NMP will experience a dramatic increase in breadth of application.
Acknowledgments
This material is based upon work supported by the National Heart Lung and Blood Institute of the National Institutes of Health as a Program of Excellence in Nanotechnology (HL080729). N. S. Lee thanks GlaxoSmithKline for their financial support through the ACS Division of Organic Chemistry Graduate Fellowship 2008–2009.
References
Zaloguj się lub utwórz konto, aby kontynuować.
Nie masz konta użytkownika?