Flash NanoPrecipitation (FNP) – Principles and Applications in Medical Imaging and Drug Delivery
Leon Z. Wang, Robert K. Prud’homme*
Department of Chemical and Biological Engineering, Princeton University, Princeton, New Jersey, US
Material Matters™, 2020, 15.3
Introduction
The principle of Flash NanoPrecipitation (FNP) was first described in 2003 by Johnson and Prud’homme as a novel method to generate highly loaded nanoparticles through a rapid mixing and precipitation process.1 FNP technology has since grown to impact a variety of fields ranging from drug encapsulation to pesticide delivery. FNP is a scalable mixing process that takes advantage of the different timescales of nucleation, aggregation, and stabilization. In the most basic form, a highly hydrophobic molecule and an amphiphilic polymer are dissolved in a water-miscible organic solvent. This organic stream is then mixed rapidly with an aqueous anti-solvent stream using specially designed turbulent mixing chambers. During this mixing, competing kinetic processes are ongoing: (1) Nucleation and aggregation of the hydrophobic active, (2) Adsorption of the steric stabilizing polymer on the aggregate, which ultimately arrests further growth. The ratio of active-to-polymer controls size; with insufficient polymer, nanoparticles grow too large, and with excess stabilizing polymer, micelles may form. At or above a threshold mixing intensity (Reynolds number, Re), where the mixing time is shorter than the nanoparticle growth time, nanoparticle size no longer depends on flowrate but only on stream composition.2 The key to the mixing is turbulence, which is scalable, and which is fundamentally different than the flows with microfluidics devices, which inherently involve low Reynolds numbers.
Evolution of Mixers and Scalability
The original mixer design comprised two opposing equalmomentum streams that collide in a cylindrical mixing volume. Using handheld syringes or syringe pumps to drive the streams, this confined impinging jet (CIJ) mixer allowed the production of small batches for rapid screening and formulation development (Figure 1). Nanoparticle size stability after formation depends on the rate of growth by Ostwald ripening, which depends on the solubility of the active in the solution phase.3 Since the CIJ mixer requires equal stream velocities, the resulting solvent:antisolvent ratio is 1:1. The antisolvent ratio, and hence active solubility, can be reduced by collecting the output of the CIJ into a quench bath. We typically use a quench bath volume to produce a final solvent concentration of 10%. Liu et al. developed a new mixer design with four mixing streams using tangential turbulent flow (Figure 1). Termed the multi-inlet vortex mixer (MIVM), each stream in the MIVM contributes independently to the micromixing process.4
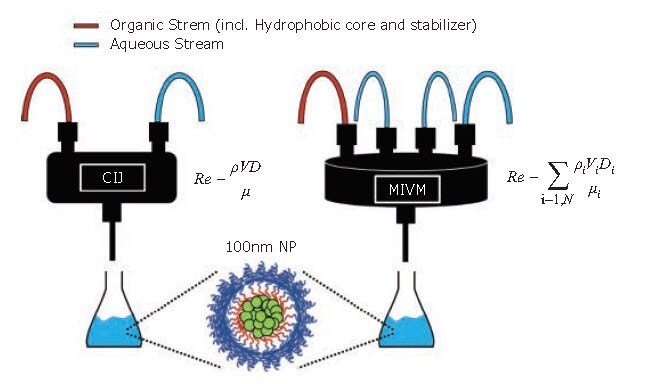
Figure 1.Schematic of the FNP process at various scales. (Left) The CIJ mixer allows for the synthesis of many small batches in rapid succession for screening formulations. (Right) The MIVM can scale up formulations to produce liters of nanoparticle solutions. (Equations for Reynolds number, Re, defined in refs. 1 and 4).
Unlike with the CIJ mixer, the momenta of the streams do not have to be matched for the MIVM, allowing for greater flexibility in nanoparticle formulations. For example, two different organic solvents can be utilized in separate streams — each containing a different API. Additionally, if sufficient dilution of the organic occurs in the mixing step, no additional quench bath is required—allowing for a truly continuous process for nanoparticle production. Markwalter et al. expanded on the MIVM design through development of a scaled-down version that required less material per batch of nanoparticles.5 Side-byside comparison between the mixing devices of different scales showed no significant variation in particle size or properties as long as sufficient mixing intensity is used.6 Comparisons between the MIVM and CIJ mixers show that nanoparticle sizes and distributions are identical above a threshold Re number (Figure 2B). Dynamic light scattering (DLS) traces and TEM images of nanoparticles formulated using the MIVM and CIJ also show no visible differences in size or properties (Figure 2A, 2C, 2D).6 This allows for a seamless development process wherein screening and small-scale formulations are performed using the CIJ mixer and then scaled up using the MIVM. A large-scale MIVM is currently being used at WuXi AppTec (Shanghai) to produce 250 nm nanoparticles of an antimalarial drug at 2 L/min in 300 L batches.

Figure 2.Comparison of nanoparticle formulations by CIJ and MIVM. (A) DLS trace of nanoparticles formulated with Vitamin E acetate core and PS-b-PEG stabilizer. Nanoparticle size is independent of the mixer type. (B) Relative nanoparticle size vs. mixing Reynolds number for CIJ and MIVM formulations. Above a threshold Reynolds number of 50000, nanoparticle size is insensitive to flow rate. (C, D) TEM images of CIJ and MIVM nanoparticles show no significant differences between nanoparticles made by the two mixer types.
Stabilizer Selection
As mentioned previously, the amphiphilic stabilizer plays a critical role during nanoparticle formation. For the majority of FNP applications, initial screening typically uses non-degradable block copolymers. Polystyrene-block-polyethylene glycol (PS-b-PEG) is a versatile stabilizer for many applications. When incorporated into a nanoparticle, the hydrophilic PEG chains form a dense outer corona around the hydrophobic core. This dense PEG layer bestows favourable biological properties such as long circulation times and mucus penetration.7 Switching some or all the PEG copolymer to polyacrylic acid (PAA) or 2-(dimethylamino) ethyl methacrylate (DMEMA) can imbue the nanoparticle with negative or positive surface charges, respectively. Since surface charge affects nanoparticle biodistribution and clearance, tuning the surface charge can help target nanoparticles to specific tissues of interest. For applications requiring biodegradable nanoparticles, the polystyrene block can be swapped out for either polylactic acid (PLA) or polycaprolactone (PCL).8 Hydrolytic degradation of these polymers allows for slow and sustained drug release from the nanoparticles, and ultimately clearance. Figure 3 contains the chemical structures of the commonly used block copolymer stabilizers. For global health applications, production costs dictate the use of lower cost materials during the formulation process. In these systems, more affordable stabilizers can be used, including hydroxypropyl methylcellulose acetate succinate (HPMC-AS), lecithin, zein, or casein.9

Figure 3.Typical polymeric stabilizers used for FNP. The relatively inexpensive polystyrene-based block co-polymers (A, B, C) are generally used for prototyping or ex-vivo applications. Biodegradable co-polymers (D, E) are used in therapeutic applications.
Applications in Oral Delivery Formulations
One application of FNP is the encapsulation of hydrophobic drugs to improve the API’s bioavailability. Clofazimine (CFZ) is a hydrophobic molecule initially used to treat leprosy but recently found to also be effective against Cryptosporidium infections, a leading cause of diarrhea in the developing world. However, CFZ, in its pure form, suffers from slow dissolution rates in the GI tract and is thus unsuitable for the fast-acting treatment required for Cryptosporidium. Flash nanoprecipitation provides enhanced solubility and improved CFZ dissolution. When encapsulated by the fast precipitation and stabilization process of FNP, the hydrophobic active molecule can be captured and preserved in an amorphous form. Additionally, the small size of FNP nanoparticles increases the specific surface area, which increases drug dissolution rate. Both factors improve the solubility and oral bioavailability of CFZ. In work performed by Zhang et al., CFZ was encapsulated into nanoparticles at very high encapsulation efficiencies (>92%) using a variety of low-cost stabilizers.9 DSC traces confirmed that encapsulation into nanoparticles preserved the amorphous state of CFZ (Figure 4A) even after processing the NPs into dry powder via spray drying or lyophilization. After incubation in simulated gastric and intestinal fluid, CFZ nanoparticles exhibited 50–90x higher supersaturation levels and complete dissolution after 6hr. Feng et al. also showed that FNP’s ability to stabilize an API’s amorphous form and improve dissolution was not unique to CFZ.10 Lumefantrine (LMN) is another hydrophobic API that is an effective treatment for malaria. Like clofazimine, lumefantrine also suffers from poor bioavailability and absorption in the GI tract. In the study, LMN was encapsulated into nanoparticles using low-cost natural polymers. Similar to the prior study with CFZ, FNP demonstrated the ability to suppress the crystallization of LMN and improved dissolution in simulated intestine fluid by over 2 orders of magnitude (Figure 4B). The amorphous nanoparticle form also decreased the difference between dissolution in fed state versus fasted state intestinal fluids. Nanoparticle formulation can decrease the sensitivity of release to environmental conditions including high temperature or humidity relative to that of traditional spray dried dispersions of the same API.11
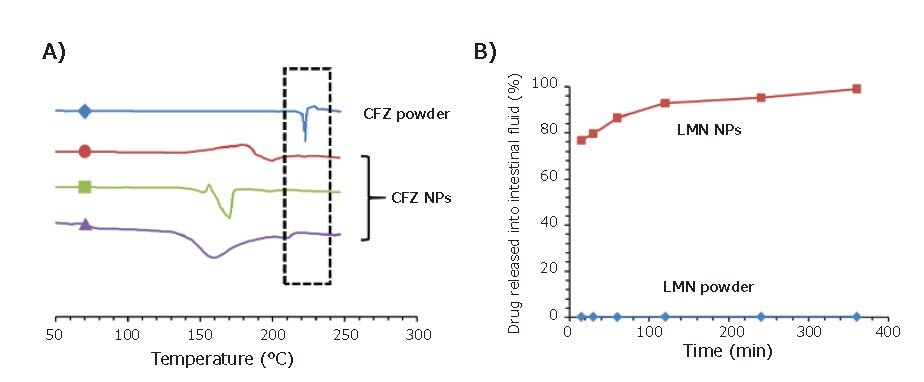
Figure 4.Applications of FNP in the encapsulation of oral therapeutics. (A) DSC trace of three clofazimine nanoparticle formulations. CFZ powder exhibits a crystallization peak at 22 °C, while nanoparticle formulations can preserve CFZ in its amorphous state. (B) Drug release assays of LMN formulations in simulated intestinal fluid. Nanoparticles exhibit close to full release after 2 hours while LMN powder exhibits <1% drug in solution.
Applications in Medical Imaging
Contrast agents have commonly been used in medical diagnostics to enable more targeted and sensitive imaging. Commonly used contrast agents, such as FDG for PET, are directly injected into the patient and thus must be water-soluble. FNP enables encapsulation of water-insoluble compounds, opening the door for a variety of previously inaccessible hydrophobic contrast agents. Furthermore, the FNP process excels at generating highly loaded nanoparticles, which can drastically improve imaging sensitivity through the concentration of contrast agents in a nanoparticle core. In one example, phthalocyanine-based dyes are encapsulated at 35% core loading using PS-b-PEG stabilizer.12 At these high core concentrations, nanoparticles can be used for contrast-assisted photoacoustic imaging. Lu et al. used photoacoustic imaging to investigate the biodistribution of nanoparticles in a mouse tumor model.13 The sharp and defined absorbance spectra of the organic dye enables multiplexed imaging, where signals are collected at various wavelengths and deconvoluted to generate a combined image. Using this technology, both nanoparticle and oxygenated/ deoxygenated blood signal can be visualized simultaneously (Figure 5A). More conventional fluorescence imaging can also be used to track biodistribution, as shown in Figure 5B. In this image of gel microparticles containing fluorescent nanoparticles, microparticle size determines capture in the lungs. Pinkerton et al. expanded the diagnostic applications of FNP to MRI through the encapsulation of iron oxide-based nanocrystals (IONC).14 Since a strong T2 MRI signal is highly dependent on IONC size and density, small clusters of IONCs were formulated into nanoparticles at up to 25% wt., using FNP in a single-step assembly process. Hydroxy-terminated PS-b-PEG was used as the stabilizer to enhance uptake by liver macrophages. In an NSCLC orthotopic mouse model, these IONCs nanoparticles were able to enhance MRI contrast of the liver and allow detection of otherwise non-visible tumor metastases (Figure 5C).
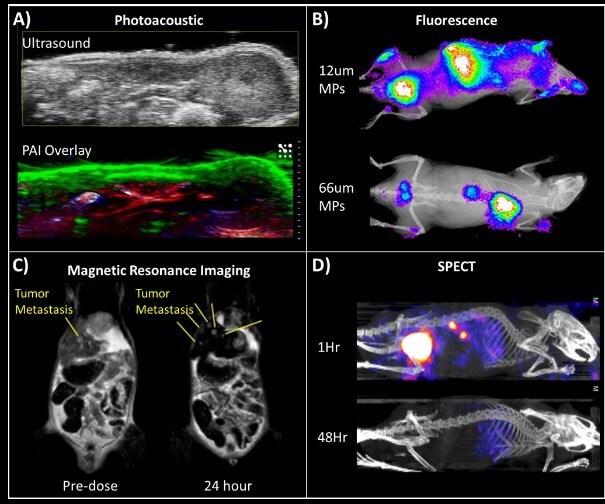
Figure 5.Biological imaging applications of FNP nanoparticles. (A) Multiplex imaging using photoacoustic tomography of a mouse model shows nanoparticle accumulation in the skin (green). Oxygenated and deoxygenated blood can also be visualized (red and blue, respectively). (B) Fluorescence of dye-encapsulated nanoparticles. Biodegradable microparticles containing these nanoparticles were injected into mice. Smaller particles accumulated in the liver while larger particles tracked to the lungs. (C) MRI imaging of liver metastases in a mouse tumor model using iron oxide-based nanocrystals (IONC) encapsulated nanoparticles. Yellow lines point to the locations of liver metastases before and after injection of IONC nanoparticles. Nanoparticles persist in healthy tissues (appearing black) — generating contrast that allows better visualization of metastasis. (D) SPECT imaging of 111In labeled nanoparticles shows biodistribution and clearance profile over time.
The use of radiotracer-based PET or SPECT imaging can further increase sensitivity and imaging resolution.15 Synthesis of nanoparticles with the ability to “load” these tracers in a fast and efficient manner is required. One approach is to conjugate a chelating molecule onto the hydrophilic end of the stabilizer. Radiotracers can then be captured into these chelators to form SPECT/PET-active nanoparticles. In a SPECT study, DPTA (diethylenetriaminepentaacetic acid) conjugated PS-b-PEG was used to chelate 111In. These nanoparticles were then used in a pharmacokinetic study to quantify the clearance mechanism and rate of clearance of nanoparticles (Figure 5D). More recently, Lu et al. demonstrated a method for radiolabeling of PEG-coated nanoparticles with 64-Cu for use in PET that has advantages over surface conjugation. Encapsulation of a phthalocyaninebased metal chelator occurs first, where the very hydrophobic phthalocyanine is in the core of the nanoparticle. At a later time the nanoparticles are “PET activated”—the PET active Cu-64 is loaded into the core through a fast and straightforward incubation step.16 This core-radiolabeling minimizes potential off-target effects from having chelators exposed on the nanoparticle surface.
Applications in Targeting to Specific Cell Types
Improved targeting of nanoparticles to desired organs or cell types can both increase the effectiveness of therapeutics and the sensitivity of diagnostic imaging. This is achieved by attaching targeting ligands to the outside of nanocarriers. These targeting ligands include antibodies, peptides, sugars, and small molecules that specifically bind to a receptor on the targeted cell. In one example, mannoside-conjugated nanoparticles were able to specifically bind to the mannose receptor of J774E macrophages (Figure 6A).17 This form of specific binding to antigen-presenting cells could expand the possibilities of nanoparticle-based vaccines. In the oncology field, FNP has been used to formulate folic acid targeted nanoparticles enabling localization to KB tumor cells (Figure 6B). In collaboration with Janssen Pharmaceutics, Elias et al. showed that scaffold proteins based on a consensus human fibronectin domain, termed Centryrins, can also be used for nanoparticle targeted therapeutics. EGFR-targeting centryrins were functionalized onto PS-b-PEG nanoparticles and successfully localized to HER2 breast cancer cells (Figure 6C).
Whereas most studies formulated the nanoparticles first, then modified the nanoparticle surface, functionalization can also occur before nanoparticle formation by directly reacting the targeting ligand onto the stabilizing polymer. Modifying the polymer prior to FNP allows for precise quantification of the percentage of functionalization on the nanoparticle surface. Furthermore, tuning of the functionalized surfaces occurs by combining modified polymer with known portions of the unmodified polymer during FNP. In one example, the hormone LHRH was modified onto the end of a PS-b-PEG polymer and then used to formulate FNP nanoparticles with various degrees of functionalization.18 In vitro results showed strong nanoparticle uptake by MS578T breast cancer cells (Figure 6D).
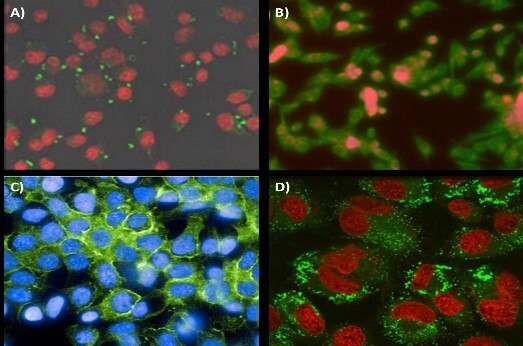
Figure 6.Targeting application of FNP nanoparticles. (A) Mannoside-targeted nanoparticle localization to the mannose receptor of J774E macrophages. Conjugation of mannoside to the PEG outer-corona ofthe nanoparticles. Nanoparticles are visualized in green while cells are in red. (B) Folic acid modified nanoparticles targeting KB tumor line cells. Nanoparticle fluorescence is shown in red while cells are stained green. (C) Centyrin scaffold targeting GFRreceptors on HER2 line cells. (D) Uptake of 80 nm nanoparticles with surface conjugated luteinizing hormone-releasing hormone (LHRH) into MS578T breast cancer cells. Nanoparticles and cells are visualized in green and red, respectively.
Applications in Encapsulating Water-Soluble Actives
While FNP works well for encapsulating hydrophobic APIs with log P >5, many less hydrophobic drugs can also be encapsulated. Currently, researchers are studying two approaches to expand the capabilities of FNP to encapsulate hydrophilic APIs. In inverse Flash NanoPrecipitation (iFNP), precipitation of a hydrophilic active occurs using an organic anti-solvent stream. Unlike a traditional FNP nanoparticle, this “inverse” nanoparticle has hydrophobic ends pointing out and hydrophilic tails pointing inward. In a second FNP coating step, a hydrophilic polymer is coated onto the iFNP nanoparticle to transfer it into an aqueous phase (Figure 7A).19 Another approach to encapsulate hydrophilic APIs involves the use of hydrophobic ion pairing (HIP). By introducing a counterion to pair with the hydrophilic API electrostatically, effectively turning off the charged groups — allowing the resulting ion-pair to precipitate during FNP. Gindy et al. utilized this method to ion-pair and encapsulate siRNA with a cationic lipid at efficiencies greater than 70% (Figure 7B).21 Ristroph et al. have reviewed the encapsulation of peptides and small molecule APIs by HIP.21
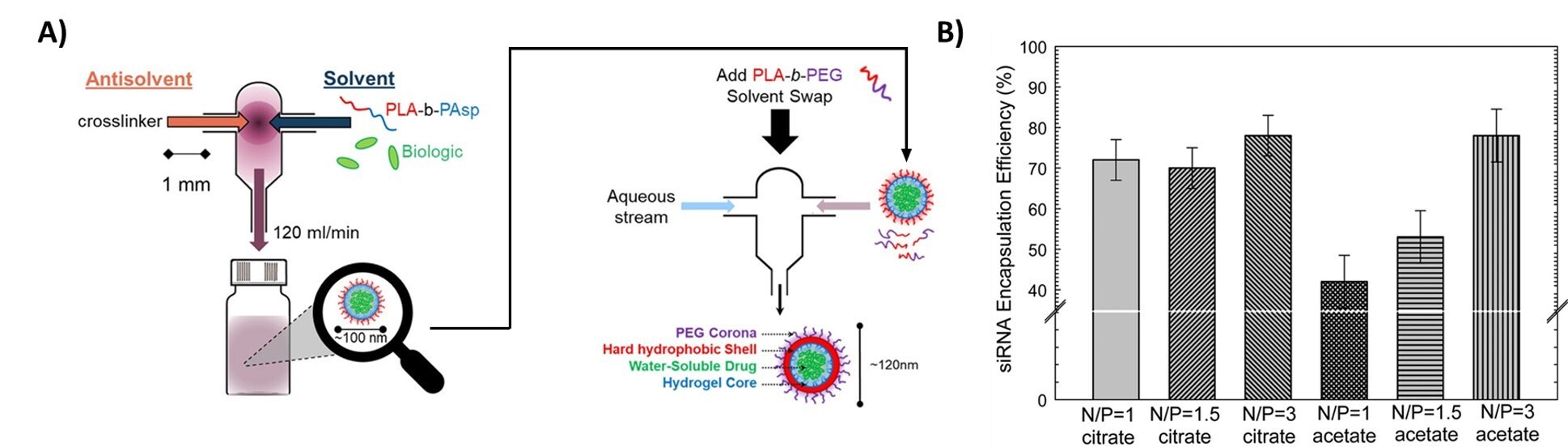
Figure 7.Encapsulation of hydrophilic actives (A) Procedure of iFNP. Precipitation of a biologic or another hydrophilic active occurs by mixing with an organic antisolvent. The resulting inverse nanoparticle is then coated with a second polymer to form a water-dispersible nanoparticle. Reproduced with permission from reference 20, copyright 2014 ACS Publishing. (B) Encapsulation of siRNA using charge pairing. Neutralization of anionic siRNA by cationic lipids allows for the formation of siRNA nanoparticles with very high encapsulation efficiency. Reproduced with permission from reference 21, copyright 2019 Royal Society of Chemistry.
Conclusion
Nanomedicine is ever-changing, with advances in formulation techniques and therapies. The FNP platform provides a technique to produce small-scale laboratory samples for research, and it has a straightforward path to large scale commercial production. It applies to a wide variety of actives from hydrophobic actives to soluble actives rendered hydrophobic by ion-pairing, to soluble oligonucleotides, peptides, and proteins by inverse-FNP. FNP, as a platform technology, is also continually evolving to both expand applications and to improve the process itself. This review presented only a selected sample of biomedical applications, but we hope it will open the door for more innovations in nanoparticle-based therapies and diagnostics. A video tutorial on the operation of CIJ and MIVM mixers for FNP nanoparticle formation has been published.22
References
Para seguir leyendo, inicie sesión o cree una cuenta.
¿No tiene una cuenta?