Electronics and Self-Assembly with Single Molecules
Kasper Moth-Poulsen, Titoo Jain, Jakob Kryger Sørensen, Thomas Bjørnholm
Nano-Science Center & Department of Chemistry, University of Copenhagen, Universitetsparken 5, 2100 Copenhagen, Denmark
Material Matters 2009, 4.3, 80.
Introduction
Single molecule electronics is the endeavour of constructing electronic circuitry with single molecules as the fundamental building block. The concept has been addressed theoretically in the 1970s1 and many experimental realizations of single molecule transistors have emerged since the middle of the 1990s.2-4 The research is motivated both by a fundamental interest for electron transport on the nanometer length scale and by a vision of developing molecular self-assembled materials as an alternative to current top-down semiconductor technologies.5,6 So far, most of the work in this field has focused on the development of test beds for the measurement of electronic transport through a single molecule. In a typical experiment, microscopic wires are fabricated by top-down lithographic techniques. The wires are later carefully broken, either mechanically or by applying a current strong enough to break the wires and leave a nanometer wide gap between the two wires.7,8 The molecule of interest is then applied to the nanogap, either via the gas phase or via solution based self-assembly. The chemical bond to the electrode material (e.g. gold) is typically formed via mercapto or amino functional groups, often referred to as a chemical “alligator clips”.9 One of the lessons learned from these studies is that atomic precision of the interface between molecule and electrodes is of paramount importance: single atom changes in contact geometry can change the conducting properties of a single molecule by up to 5 orders of magnitude.10 Modern silicon based computer chips consist of 106-109 active components, yet molecular electronics with single molecules are at a stage where it is challenging to make reproducible measurements on the single device level. Major improvements in device fabrication methods and in the way the molecules are contacted to the electrodes are therefore needed. One of the challenges in the field is therefore to develop methods that allow for the integration of multiple molecular components in a single experimental realization. In this report we present some of the emerging methods for self-assembly of single molecule circuitry together with concepts for the chemical control of the interface between molecule and electrode based on the recent work at the Copenhagen University Nano-Science Center.4,10-16
Self-assembly at the Air-Water Interface
Self-assembly at the air-water interface, facilitated using the so-called Langmuir-Blodgett (LB) technique, is a well established method that has revealed much knowledge of the inherent behavior of both molecules and nanoparticles. The technique involves the use of amphiphilic molecules that form a monolayer at the water-air interface. By adjusting the surface tension the molecules are forming 2-dimensional architectures which are readily studied by a handful of techniques such as X-ray reflectometry, grazing incidence X-rays, or simply by scanning probe techniques. Gold nanoparticles have become popular building blocks for various nanoscale assemblies.6,16 Here, 1-2 nm gold nanoparticles were prepared by the so-called Brust method, which is the reduction of Au(III) by NaBH4 in a two phase system in the presence of a protecting thiol, typically dodecane thiol (471364).17 In an effort to construct gold nanowires by self-assembly the gold nanoparticles were spread on a water surface in a Langmuir-Blodgett trough. When co-spread with a suitable surfactant, such as DPPC18 (D206555) or ambipolar polymers,12 the gold nanoparticles are organized into wires or maze structures (Figure 1). Subjecting the assembled wire structures to solutions of oligo(phenylene-vinylene)s (OPVs) allows for electronic characterization, and therefore an estimate of the single molecule conductances.13 The prospects of using the air-water interface for the self-assembly of inorganic nanoparticles is further exploited in a recent review by Tao et al.19
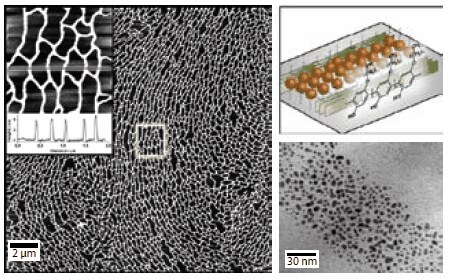
Figure 1. Self-assembled maze structures of gold nanoparticles. The structures were assembled at the air-water interface by co-spreading gold nanoparticles together with an amphiphilic polymer. AFM overview of the maze structure (left), illustration of the self-assembly process (top right) and high resolution TEM of a wire consisting of gold nanoparticles.12
Self-assembled Nanogaps with a Single Molecule Incorporated
One of the important challenges in molecular electronics is the preparation of a 1-2 nm nanogap with a single molecule situated in the nanogap. In a recent report, gold nanorods were used to bridge the gap between the molecular length scale (1-2 nm) and the micrometer length scale, which is readily accessible by simple lithographic techniques.11 Gold nanorods have recently attracted large interest due to their ease of synthesis, unique shape dependant optical properties, and possible biochemical imaging20 and medical applications.21 The most widely used preparation method is the seed-mediated and surfactant assisted synthesis, first reported by Murphy and co-workers.22 In brief, citrate-stabilized gold nanoparticle seeds are prepared by the reduction of HAuCl4 (484385) with NaBH4 (480886) in an aqueous citrate solution. The seeds are then added to a growth solution containing the surfactant CTAB (H9151), HAuCl4 and ascorbic acid (A5960). Depending on the specific growth conditions and purification schemes, rods with aspect ratios from 4 to 25 can be prepared.23,24 Important factors for the successful synthesis are the use of the correct type of CTAB surfactant25 ( H6269), precise temperature control and clean glassware. Gold nanorod dimers can be prepared by addition of a water soluble thiol end-functionalized polyethylene glycol (HS-PEG-SH) to the gold nanoparticle seeds before they are exposed to the growth conditions. By carefully tuning the concentration of HS-PEG-SH the reaction conditions are optimized to yield a high degree of gold nanorod dimers. By diluting the HS-PEG-SH concentration further, one can, to a high degree, expect that a single molecule is acting as the linker between two nanorods (Figure 2).11

Figure 2. Scheme depicting the synthesis of linked gold nanords (left). TEM images of linked gold nanorods at different magnifications (right).11
In another experiment (Figure 3), the electronic properties of the gold rods were tested. First, gold nanorods were self-assembled to gold nanoparticle-coated tin oxide nanowires. The assembly was facilitated using thiol end-capped oligo(phenylenevinylene)s (OPVs) which are interesting test molecules exceeding low tunneling barriers26 and widely used in molecular electronic experiments.4,10 Second, the electronic properties of the OPV molecule were probed by conducting atomic force microscopy (C-AFM). The conductive AFM tip was placed directly on the gold nanorod as one electrode, while the much larger tin oxide nanowire acted as the second electrode, which was easily connected to close the electronic circuit around the molecule (Figure 3). I-V characteristics revealed signatures similar to those previously obtained for OPV molecules, thus also confirming that gold nanorods may be promising candidates for bridging the gap between the molecular and micrometer length scale.

Figure 3. Artistic impression of the measuring of a single or a few molecules via a conducting AFM tip (left) and AFM picture (right) of gold nanorods self-assembled to tin oxide nanowires mediated by thiol end-capped oligo(phenylenevinylene) molecules.14
Synthesis of a Conducting Molecule with Well Defined Contact Geometry
Control of the contact between a molecule and the electrode surface has proved challenging since minute changes in atomic position of the binding thiol or the atomic structure of the surface may lead to large difference in the electronic properties.27-29 In an attempt to circumvent the challenges discussed above, C60 was introduced as an alternative anchoring group. The C60 molecule can create a strong contact to the surface through multiple connection sites, thus rendering the details of the contact site less important since the limiting tunneling barrier is now moved from the interface between molecule and electrode to well defined chemical bonds inside the molecule. As a consequence, the detailed contact geometry can then be controlled by chemical synthesis of C60 derivatives (Figure 4). The first electronic measurements taking use of this concept was described by Martin et al.15 who performed single molecule measurements using the break junction technique, and found that one of the dominant features of the molecule was extremely high stability of the single molecule junctions - even at room temperature.
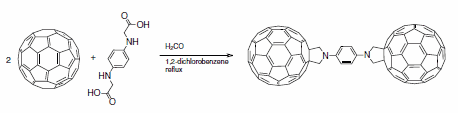
Figure 4. Synthesis of a Würster’s blue derivative with C60 alligator clips.15
The C60-anchored molecule (1,4-bis(fullero[c]pyrrolidin-1-yl)benzene) was synthesized via [2+3]cycloaddition reaction of an in situ generated azomethine ylide to a fullerene carbon-carbon double bond. This reaction is known as the Prato-reaction.30 In brief, N,N’-(1,4-phenylene) bisglycine and paraformaldehyde (16005) was sonicated in 1,2-dicholorbenzene (240664) and added to a solution of C60 in 1,2-dichlorobenzene. The reaction mixture was refluxed for 6 hours yielding the black target molecule in 28% yield after purification (Figure 4).15
Conclusion
In order to be able to study the intrinsic charge transport mechanisms in individual molecules, one of the main challenges is to circumvent problems associated with the specific position of the bonds between electrodes and molecules. A strategy for predefining the metalmolecule interface, and thereby achieving atomic scale precision, by the introduction of new C60-based anchoring groups have been presented. Another challenge is the fact that only one molecular junction is realized per device, hence the realization of molecular circuitry with numerous individual addressable components is highly desirable. Alternative routes that may help overcome these challenges are the use of chemical bottom-up fabrication techniques such as the Langmuir-Blodgett technique or other self-assembly based methods that may aid in the development towards complex circuitry. One possibility is the bottom-up synthesis of electrodes (gold nanorods) with molecules incorporated directly in the nanogap and prepared by the use of simple aqueous solution-based chemistries.
References
Zaloguj się lub utwórz konto, aby kontynuować.
Nie masz konta użytkownika?