Quantum Dots: An Emerging Class of Soluble Optical Nanomaterials
Professor Philippe Guyot-Sionnest
Depts. of Physics, Chemistry and The James Franck Institute, University of Chicago
Material Matters Volume 2 Issue 1
Colloidal Quantum Dots (QDs) offer a striking visual demonstration of Quantum Mechanics. Atomic structures and sizes of the QD nanocrystals can be clearly seen in electron micrographs. When illuminated by an ultraviolet light, the nanocrystal solutions glow in vibrant and distinct colors. It is impressive to see the direct correspondence between the colors, from red to blue, and the increasing sizes of the nanoparticles (Figure 1). This compelling demonstration of Quantum Mechanics is the result of many years of advances in synthetic colloidal chemistry, motivated by developing technological interest in QD materials. This article will highlight the convergence of physics, chemistry, and materials science that made this emerging class of materials possible.

Figure 1.Top panel: Schematic image (left) of a QD nanocrystal core surrounded by solubilizing ligands; TEM image (right) of a dried film of monodisperse QDs packed in a hexagonal array. A high-resolution picture of a single QD showing atomic planes is shown in the inset. Bottom Panel: Fluorescence display of colloidal solutions of CdSe/ZnS Quantum Dots.
QD Physics
An electron confined in a potential energy box is the most elementary problem we tackle when first exposed to quantum mechanics. We learn that the electron kinetic energy increases as the box gets smaller. This is the result of the uncertainty relation DxDp ; ħ/2, one of the fundamental principles of quantum mechanics. Using a box length L = Dx and electron momentum p = mv, the minimum kinetic energy of the electron is no longer zero but a finite value E = ħ2/2mL2 (with Plank’s constant ħ and electron mass m). This expression is the basic principle behind the effect of size L on the color of semiconductor quantum dots. In addition, the defining property of a semiconductor is that there is an energy gap (band-gap) between a filled continuum of electron energy states (valence band) and an empty continuum of states (conduction band). A nanocrystal is a small semiconductor box and therefore the band-gap between the valence state and the empty state increases as the box gets smaller (Figure 2).

Figure 2.Schematic of the effect of the decreased size of the box on the increased energy gap of a semiconductor quantum dot, and the resultant luminescent color change from bulk materials (left) to small nanocrystals (right).
The above expression, which uses the free electron mass m, predicts very small effect of box size on the semiconductor band-gap at the nm scale. The problem is the use of the free electron mass. In crystalline solids, electrons behave as quantum mechanical waves, “sneaking though all the ions” as Felix Bloch put it, so that instead of the free electron mass m, they behave as if they had an “effective mass” m*.1 These effective masses were measured by cyclotron resonance starting in the mid-1940s. In some semiconductor materials, the effective masses can be quite small, a tenth or a hundredth of the free electron mass. For example, for CdSe, the effective mass is 0.13 the free electron mass. The smaller mass makes the semiconductor materials much more sensitive to the size of the box at the nm scale, and this has dramatic consequences, the most obvious being the increasing energy gap for smaller nanocrystals.
The particle in the box concept applied to semiconductors first surfaced in the 1960’s at IBM.2 Molecular Beam Epitaxy allowed building thin layers of materials with atomic precision thickness, such as GaAs/AlxGa1-xAs heterostructures. These one-dimensional electron boxes were called Quantum Wells. One purpose of that work was to “engineer” the lowest energy transitions of semiconductors by choosing the well size. Another consequence of the spatial confinement is to increase the probability of recombination of an electron in the conduction band and a missing electron (hole) in the valence band. This makes Quantum Wells excellent tunable diode lasers. Today, most semiconductor lasers used in optical communications and optical data storage systems (such as DVDs) are based on Quantum Well heterostructures
QD Chemistry
Quantum Dots extend the idea of quantum confinement to three dimensions, and colloidal chemistry is a very natural way to make nanoscale semiconductor particles. The topic originated in the early 80’s, when papers in the Russian literature discussed the size-dependent absorption spectra of colloidal semiconductor nanocrystals grown in molten transparent glasses or crystals.3,4 Fast-forward in time, and we have colorful luminescent solutions of QD colloids, covering the near UV to near IR, which can be purchased from a growing number of companies around the world.
This achievement arose from many synthetic advances over the past twenty years. At first, the chemistry community explored colloidal semiconductor nanocrystals synthesized by aqueous and ionic chemistry. Part of the motivation, following the first oil crisis, was the development of nanocrystals for solar energy conversion and photocatalysis. Besides using quantum confinement to optimally tune the absorption wavelength and redox potential of the nanocrystals, it was felt that the high surface area of the particles would improve collection efficiency or surface reaction yields.5,6,7 However, the aqueous colloidal synthesis made materials with poor size distribution and low fluorescence efficiency. While monodispersed colloidal growth was well known for amorphous sub-μm sulfur or silica particles, as in the earlier works of La Mer8 or Stauber,9 the growth of crystalline monodispersed nm-sized colloids turned out to be more difficult. Further improvements in material properties waited for the development of a better synthetic approach.
In 1993, new scientific breakthroughs radically shifted the synthesis of semiconductor nanocrystals toward using high-temperature organic solvents. With pyrophoric metalorganic complexes that rapidly decompose, and long chain surface-passivating ligands, it became possible to achieve fast nucleation and slow growth of the nanocrystals, while maintaining a high enough temperature to anneal defects.10 This resulted in vastly improved crystalline nanoparticles with high monodispersity and strong confinement. These new materials, at first mostly CdSe, led to many advances in the characterization of the electronic properties of the colloidal Quantum Dots (Figure 3). Using this route, colloidal quantum dots of other materials, for example II-VI and III-V semiconductors, could also be synthesized.
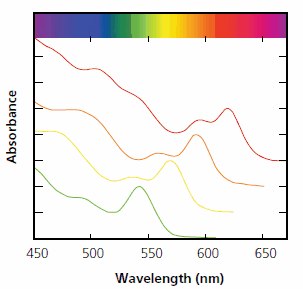
Figure 3.Absorption spectra of four CdSe colloidal quantum dots with distinct diameters from 3.5 nm to 5 nm. The synthesis with cadmium acetate and selenium requires no glove box and produces quality nanocrystals without post-reaction processing. Bulk CdSe is gray and absorbs below 720nm. The structure in the spectra is due to optical transitions between discrete (quantized) energy states within the valence and conduction bands of the nanocrystals.
An important feature of the small nanocrystals is the great proportion of surface atoms. If the goal is to achieve a good and stable luminescence yield, the surface must be inert to the excited electrons. In other words, there can be no surface states to trap the electron. Materials with a simple CdSe core had poor photoluminescence and moderate photostability. In 1996, this drawback was solved by growing a shell of ZnS,11 a higher band gap semiconductor, around the CdSe core. The CdSe/ZnS core/shell Quantum Dots, and subsequent core/shell systems afforded strong and sturdy photoluminescence which further generated interest for these materials. Still, the use of pyrophoric metal organic precursors was a major inconvenience. In 2000, the introduction of stable ionic precursors, or “green” precursors,12 dramatically reduced the psychological barrier and some of the potential hazards of the synthesis of II-VI nanoparticles. This was achieved without losing any of the unique physical properties of materials prepared by earlier synthetic methods, as shown in Figure 3. This approach, since then demonstrated for many II-VI and IV-VI semiconductors, has led to an explosion in the number of groups synthesizing and investigating semiconductor nanocrystals. The new methods to synthesize the nanoscale colloids, coupled with visualization by Transmission Electron Microscopy (TEM), are now facilitating serendipitous discoveries of materials with novel shapes. The last five years produced about 5 times more scientific publications on colloidal quantum dots than the previous twenty years combined. What has sustained the growth of research activity is the potential of these novel colloidal materials for improving
QD Applications
To date, the most successful application is the use of colloidal Quantum Dots as biological tags, a strategy first demonstrated in 1998.13,14 It turns out that core/shell Quantum Dots can be made more photostable (long-lived) than existing molecular dyes. They also exhibit narrower luminescence, a broader and continuous absorption spectrum, and can be tuned to the near infrared with still high quantum efficiency. For all these excellent reasons, there is a considerable level of activity to modify the surface of the Quantum Dots, rendering them specific for imaging particular biological targets. A more traditional area of application of semiconductors is electro-optic devices, such as Light-Emitting Diodes (LEDs), Photovoltaics (PV), and lasers. The colloidal nature of Quantum Dots opens up the route to cheap and large-scale processing of semiconductor thin films using spraying or printing methods. The challenge is to combine their good optical properties with equally good electronic properties. Great progress is being made. For example, a few years after the inception of Organic LEDs, it was suggested that Quantum Dots could also be used to make LEDs15 with similar potential benefits for large area and flat panel displays. Recently, LEDs with efficiencies of a few percent, sufficient for commercial interest, have been achieved.16
Another possible application of Quantum Dots, highlighted by recent energy concerns, is in solar energy conversion. This was one of the early motivations for these materials and is again actively researched by many groups. To print large area solar cells, semiconductor colloids are a valid starting point. It is also a possibility that the “Quantum Dot” nature of the material may be an advantage. Selecting particle sizes would allow optimal tuning of the absorption band gap to match the solar spectrum, and it is also believed that it might help to extract the energy from short wavelength photons.17
There are other promising applications presently pursued with lesser vigor such as using colloidal Quantum Dots to make semiconductor lasers. Quantum Dots have long been proposed as the best semiconductor materials for highly efficient lasers,18 and Molecular Bearm Epitaxy has been used to make electrically driven heterostructure Quantum Dot lasers for nearly a decade. However, the colloidal Quantum Dot packing density achieved so far in these devices is too low to provide a net advantage over existing Quantum Well lasers. With dried films of close-packed colloidal Quantum Dots (Figure 1), the packing density can be increased significantly showing great promise for improved lasers. At present, photopumped lasing has been demonstrated,19 and electrically pumped lasing is a challenge for the future. Another possible display application, with low current requirements, would use the color changes of redox-active Quantum Dots. Adding two electrons to a Quantum Dot fills the lowest unoccupied energy state in the conduction band, which changes the absorption of the dot, thereby changing its color.20 This flexibility of changing Quantum Dot colors, by engineering their redox potentials, makes this application possible.
Colloidal Quantum Dots have a particular niche as optical materials in the infrared. Indeed, in the visible and UV spectral range, organic dye molecules can have near 100% photoluminescence efficiency and Quantum Dots struggle to compete. However, in the near-infrared QDs win easily. This is because organic dye molecules have high frequency vibrations that couple strongly with electronic transitions. Thus the quantum yield of dye molecules falls below 1% at wavelengths around 1 μm and organic dyes provide no detectable photoluminescence beyond 2 μm. Inorganic Quantum Dots made from semiconductor materials with heavy atoms and infrared band gaps have very low vibrational frequencies and some, such as PbSe, can be excellent emitters in the near infrared. Quantum Dots can be strong emitters in the mid-infrared as well, and there has been progress in this direction in recent years.21 Potential applications could include earth to satellite communications through the atmosphere transparent bands with wavelengths of 3-5 μm and 8-10 μm.
There are many interesting chemical challenges to further applications of colloidal Quantum Dots. Besides size, shape, and surface control, semiconductor composition is an issue. For example, doping n- or p-type impurities in Quantum Dots is important for electronic applications. Adding magnetic impurities inside a small nanocrystal is a way to strongly enhance the magnetic response of the nanocrystals.22 Research is intense in this direction, with the goal being to make new magneto-optic and spintronic materials. It is clear that colloidal Quantum Dots have a bright future as specialty optical materials. They are one example of a general trend toward rational design of nanomaterials where known principles of physics are fused with modern chemical techniques to create nm-sized materials with properties optimized for targeted applications.
References
Para seguir leyendo, inicie sesión o cree una cuenta.
¿No tiene una cuenta?