Biodegradable Polyester Based Nanoparticle Formation by Miniemulsion Technique
Anna Musyanovych, Katharina Landfester
Max Planck Institute for Polymer Research
Material Matters 2012 v7, n3
Introduction
Aliphatic polyesters such as polylactide, poly(lactide-co-glycolide) and polycaprolactone, as well as their copolymers, represent a diverse family of synthetic biodegradable polymers that have been widely explored for medical uses and are commercially available.1 These polyesters have excellent tissue compatibility and safety profile, both of which are critical for their successful utility in the human body. Moreover, these polymers have been approved by the United States Food and Drug Administration (FDA) and European Medicine Agency (EMA) for many biomedical applications such as drug delivery devices, sutures and implants. Biodegradable polymer nanoparticles have been extensively explored for delivery of both hydrophobic and hydrophilic drugs to improve bioavailability, bioactivity and control delivery. This article explores the broad range of polyester-based biodegradable polymers utilized in drug delivery and describes their physiochemical properties, methods utilized to form polymer nanoparticles, and the degradation processes these materials undergo in biological environments.
Biodegradable Polyesters
Polylactide is a thermoplastic biodegradable polyester derived from renewable resources. The properties of polylactide are dictated by its enantiomeric form. Lactic acid (or lactide) monomer is a chiral molecule with two possible enantiomers, D and L, and therefore, "polylactide" exists in the following forms: poly(L-lactide) (PLLA), poly(D-lactide) (PDLA), and the mixture of both, poly(D,L-lactide) (PDLLA). Polylactides with PLLA content >90% tend to be crystalline; whereas PDLLA is an amorphous polymer due to the random positions of its two isomeric monomers within the polymeric chain. Semicrystalline polylactide is generally preferred to the amorphous form when higher mechanical properties are required (tensile strength of 50-70 MPa). Polylactides have a glass transition temperature (Tg) and melting temperature (Tm) in the range of 50-65 °C and 175-180 °C, respectively, with higher L-lactide content contributing to increased transition temperature values.
Poly(lactide-co-glycolide) (PLGA) is a random copolymer with physical and mechanical properties that can be easily tuned by altering the lactide to glycolide ratio. The Tg of PLGA copolymers is reported to be above the physiological temperature (>37 °C) and hence glassy in nature. PLGAs comprised of 25-75% lactide are amorphous and hydrolytically unstable, thus they are very promising candidates for drug delivery applications. Furthermore, PLGA has been used in tissue engineering due to its excellent cell adhesion and proliferation properties. A recent, comprehensive review by Danhier et al. explores PLGA-based nanoparticles for biomedical applications.2 Modified PLGA nanoparticles are able to cross the blood-brain barrier and have a potential use in drug delivery to the central nervous system.
Poly(ε-caprolactone) (PCL) is a semicrystalline polymer with a low tensile strength (~23 MPa), low Tg (~-60 °C) and Tm (~55-60 °C). Under physiological conditions, PCL degrades slower than polylactide and, therefore, has numerous applications in tissue engineering as an elastic material for preparation of the long-term implantable devices.3,4 PCL-based nanoparticles are also widely explored for utility in controlled drug delivery systems.5
Polymer Nanoparticle Formation Methods
Nanoparticles formulated from biodegradable polymers are of great interest for drug delivery purposes. A wide variety of hydrophilic and hydrophobic drug molecules such as DNA, proteins, etc., can be encapsulated by biodegradable polymers and delivered to specific organs or cells in nanoparticle form.6 In the past few decades, several different preparation techniques have been published describing the formulation of polyester-based particles (or capsules). The choice of a particular approach mainly depends on the physiochemical properties of the polymer (i.e., solubility and molecular weight) and the incorporated biologically active material (i.e., hydrophobicity/hydrophilicity of the drug and its sensitivity to the solvent). Currently, the most popular methods are emulsion/solvent evaporation or diffusion, double emulsion, flash nanoprecipitation and the salting out procedure, all described below.
The emulsion/solvent evaporation method involves the preparation of an oil-in-water emulsion, where a small quantity of nonpolar organic solvent containing polymer and hydrophobic biological material (e.g., drug) (oil phase) is added to a polar solvent (water phase), containing a stabilizer.7-9 The most common stabilizers are hydrophilic molecules such as poly(vinyl alcohol), polysorbates (TWEEN®), poly(acrylic acid), poloxamers (or Pluronic®) and sodium dodecyl sulfate. Stable nanoparticles are formed in the aqueous phase by organic solvent evaporation, under increased temperature or reduced pressure. In the emulsion/diffusion method, the oil phase consists of polymer dissolved in a partially water miscible solvent (e.g., ethyl acetate, propylene carbonate, etc.).10,11 The addition of a certain volume of water to the oilin- water emulsion induces a change in the equilibrium of the system and causes the partially water miscible solvent to diffuse from the droplets into the aqueous phase. This reduces the polymer′s solubility and results in particle formation.
Another approach is the double-emulsion, water-in-oil-in-water, method.12,13 The main benefit of the double-emulsion method is its ability to efficiently encapsulate hydrophilic drugs. In this approach, the drug, dissolved in water, is added to an organic solvent containing polymer, forming a water-in-oil emulsion. Then a small quantity of this initial emulsion is added to a second aqueous phase containing an emulsifier, such as PVA, to stabilize the particle. Polymer nanoparticles containing hydrophobic drugs are obtained by evaporation of the organic solvent. Nanoprecipitation (or solvent displacement) method, utilizes interfacial polymer deposition to form nanoparticles.14 In this process, polymer and a lipophobic drug are dissolved in a water miscible solvent, semi-polar solvent such as acetone and this solution is added to an aqueous solution containing a stabilizer. Solvent diffusion gives rise to polymer precipitation on the interface between the aqueous phase and finely dispersed oil droplets, resulting in the formation of solid particles. Finally, the salting out method is an oil-in-water emulsion comprised of a primary aqueous phase containing stabilizer and a high concentration of salt (e.g., magnesium chloride hexahydrate) and polymer dissolved in a water miscible solvent, such as acetone or tetrahydrofuran.15 Due to the presence of a high concentration of salt, there is no diffusion of the solvent into the aqueous phase. Fast addition of a large amount of water to this oil-in-water emulsion reduces the ionic strength and leads to the migration of the water soluble organic solvent into the aqueous phase, inducing formation of solid particles. In this method, particles must be purified to remove residual salt and solvent prior to use. The resulting size of nanoparticles/capsules depends strongly on the preparation process, as well as on the molecular weight and hydrophilic/hydrophobic nature of the polymer used.
Combined Emulsion/Solvent Evaporation and Miniemulsion Techniques
By combining emulsion/solvent evaporation and miniemulsion techniques, it is possible to produce biodegradable nanoparticles with a controlled size and narrow size distribution.16,17 The process consists of an emulsion comprised of water-immiscible organic phase and aqueous phase containing polymer and hydrophilic stabilizer. A miniemulsion is achieved using high shear rates to form monodisperse nanodroplets ~50-500 nm in diameter. Subsequently, the organic solvent is evaporated from the system causing the polymer to precipitate within the spherical nanoreactor forming size-controlled solid particles. The formation process of these well-defined polymer nanoparticles is illustrated in Scheme 1.
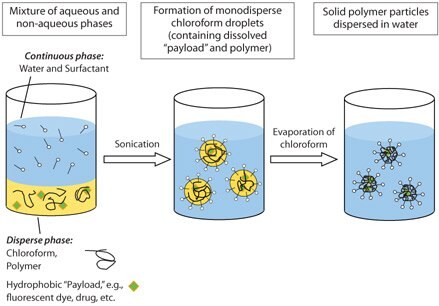
Scheme 1. Formation of biodegradable nanoparticles in the oil-in-water miniemulsion method.
A series of five different polymers were used to produce nanoparticles stabilized with the anionic sodium dodecyl sulfate (SDS) from Scheme 1, shown below in Table 1. The effect of molecular weight and polymer type used to prepare polymer nanoparticles on particle size and size distribution were studied. Particles were prepared using 72 mg of SDS and 0.3 g of polymer.
The size of nanoparticles prepared using these polymers range from 76 to 165 nm in diameter. The smallest particles were obtained with PLGA and largest with PCL-2. In general, higher molecular weight results in ability to form larger particles by this technique, using SDS as a stabilizer (121 nm for PLLA-1 and 106 nm for PLLA-2). The morphology of the polymer nanoparticles was studied by scanning electron microscopy (SEM). All particles display spherical shape with narrow polydispersity. PLLA-1 particles are shown in Figure 1, as a representative example.
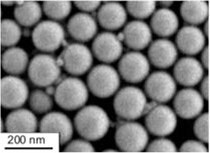
Figure 1. SEM image of PLLA-1 showing spherical shape and narrow polydispersity.
The loading efficiency of polymer particles was studied using a hydrophobic fluorescent dye marker (N-(2,6-diisopropylphenyl)-perylene-3,4-dicarbonacidimide) (PMI) as a model system. A series of polymer nanoparticles containing 0.5-2.98 mg of PMI per g of polymer were prepared. However, after purification of the particles the final concentration of encapsulated PMI was determined to be ~0.7 mg/g of polymer, regardless of the polymer used. Due to the presence of negative surface charges, these particles were employed as templates for the biomimetic mineralization of calcium phosphate.18
Nanoparticles Degradation Processes
Degradation of aliphatic polyesters follows one or more mechanisms including chemical hydrolysis, microbial, enzymatic, and thermal degradation.19 Hydrolysis begins with water uptake, followed by hydrolytic cleavage of ester bonds. Hydrolysis in polymers can be influenced by various factors such as chemical structure (e.g., crystallinity and hydrophobicity), molecular weight and its distribution, purity, morphology, processing method, and storage conditions (e.g., temperature, pH, presence of salts, etc.).20 Polycaprolactone degrades in at least two stages. In the first stage, non-enzymatic bulk hydrolysis of the ester bond occurs, which is autocatalyzed by carbon end groups of the polymeric chain. In this stage, PCL-devices maintain their shape and weight. The second stage starts when the PCL degrades to molecular weight fraction of ~5,000 g/mol. At this point, the rate of chain scission decreases and weight loss continues by diffusion of oligomers out of the polymer matrix. PLGA polymers are degraded into lactic and glycolic acids, which are eliminated from the body as carbon dioxide and water.
Degradation of particles PLLA-1, PLLA-2, PCL-1, PCL-2, and PLGA was estimated from the rate decrease in the weight average molecular weight (Mw). Taking into account the ultrasonication (US) step during the miniemulsion formation induces the polymer degradation, Mw of the final particles prepared without ultrasonication was also studied. Normalized values of the weight loss as a function of storage time are shown in Figure 2.

Figure 2. Degradation as a function of polymer type and molecular weight, measured before ultrasonication (US), immediately after US, then 45, 150, and 285 days after US.
All particles formed via ultrasonication showed a reduction in the polymer molecular weight, especially in the case of PCL where the molecular weight reduction was 70-75 wt. %. Polymers with high initial molecular weights (PLLA-1 and PCL-1) showed more degradation by ultrasonication than polymers with shorter chain length (PLLA-2 and PCL-2). The difference in weight loss is attributed to enhanced thermal degradation of polymers containing more chain entanglements induced by the high shear of the system during ultrasonication. During aging, molecular weight decreases rapidly as a result of random hydrolytic cleavage of ester bonds. The degradation rate of the amorphous PLGA particles was slightly faster compared to the other polymers, due to its higher permeability. Over a period of 12 months, the molecular weights of PLLA, PCL, and PLGA nanoparticles decreased by 51, 78, and 54 wt. %, respectively; whereas, particle size did not change significantly, even though molecular weight decreased.
Conclusions
Tremendous efforts have been put into research of biodegradable synthetic polyesters. Polyester-based nanoparticles remain one of the most versatile and promising class of biomaterials that can be processed to meet specific requirements in biomedical applications. Forming polymers into nanoparticles allows control over the types of drugs that can be encapsulated, such as hydrophilic drugs, hydrophobic drugs, vaccines, proteins, and nucleic acids.2 Incorporation of different anticancer drugs, such as paclitaxel, cisplatin and hypericin in polyester-based nanoparticles (with a size range between 50 and 500 nm) strongly enhances their antitumor efficacy compared with free drugs. This enhancement is believed to be caused by the polymeric material efficiently protecting the encapsulated agent from enzymatic degradation. In addition to providing protection, nanoparticles can be used to deliver drugs at interfaces. For example, polyester-based nanoparticles can deliver growth factors to tissue engineered vascular grafts, and PLGA nanoparticle-coated stents can effectively deliver genes or drugs to vessel walls. Selection of an appropriate polymer composition and molecular weight affords the degradation kinetics and release profile of the encapsulated agent to be tuned over a broad range. Further studies on particle shape, formulation, and functionalization will lead to continuous developments in targeted delivery and theragnostics to ensure success in production of new and improved biomaterials.
References
若要繼續閱讀,請登入或建立帳戶。
還沒有帳戶?