Functional RAFT Polymers for Biomedical Applications
M. Shahinur Rahman, Sebastian Grajales
Material Matters, 2016, 8.3, 96
Introduction
Reversible addition–fragmentation chain transfer (RAFT) polymerization is rapidly moving to the forefront in construction of drug and gene delivery vehicles. The RAFT technique allows unprecedented latitude in the synthesis of water soluble or amphiphilic architectures with precise dimensions and appropriate functionality for attachment and targeted delivery of diagnostic and therapeutic agents. To date, efforts have focused on the use of RAFT polymerization for generating block copolymer micelles, vesicles, star polymers, nanoparticles, and capsules as potential advanced drug carriers and also polymer-drug conjugates as prodrugs (Figure 1).1 This review focuses on the overview of the RAFT process, selection of appropriate RAFT agents, and its potential for building tailor-made (block) copolymers, functional polymers, and polymers with a wide range of biological recognition.
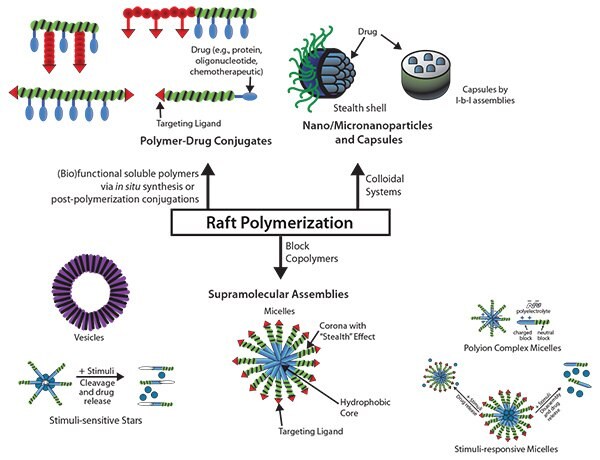
Figure 1.Examples of Controlled Drug Release Systems Generated by RAFT-Polymers: supramolecular assemblies including micelles, vesicles, and stars; Nanoparticles, microparticles, and capsules; and polymer-drug conjugates. Adapted from Liu, et al., Reference 1.
RAFT Polymerization
Controlled radical polymerization (CRP) techniques have been previously reviewed in articles,2,3 books,4 and a guide.5 These techniques rely upon quickly establishing an equilibrium between an active, propagating polymer chain and a dormant moiety. The RAFT process generates polymers with precisely controlled structural parameters such as random, block, gradient, grafted, and star copolymers.6-9 The advantages of RAFT polymerizations include the ability to operate in a wide variety of reaction conditions and solvents. In RAFT polymerization, chain transfer agents (commonly referred to as CTAs or RAFT agents) create a reversible addition/ fragmentation equilibrium (Scheme 1). In this process, the initial generation of a radical produces a propagating polymer chain. This polymer chain will (ideally) quickly react with a RAFT agent, which will functionalize the end of the chain with a RAFT moiety, therefore causing dormancy. The remaining moiety of the original RAFT agent will then initiate a new second polymer chain. This second chain will eventually react with a RAFT-functionalized polymer chain, creating an environment in which polymer chains are alternating between being dormant and active and therefore growing at similar rates. This ideally yields polymer chains that are all comparable in chain length.
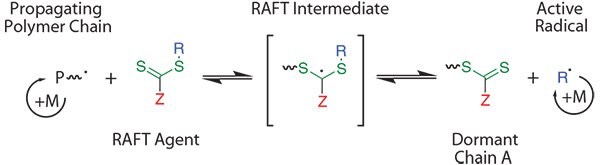
Scheme 1.Simplified mechanism of a RAFT polymerization. Essentially, a radical (in the form of a propagating polymer chain) reacts with a RAFT agent, which can reversibly fragment to create a new active radical (in blue). As the radical reacts with nearby monomer units, “R” will represent a second propagating polymer chain. This results in the thiocarbonylthio group (in green) transferring from chain to chain acting as a “protecting group.” The Z group (in red) dictates which types of monomers can be effectively controlled.
While RAFT is versatile and there are RAFT agents available for a wide variety of monomers, the limitation of RAFT is that there is not any one RAFT agent capable of controlling all types of monomers. RAFT agents must be selected based upon the corresponding monomer type used in order to obtain the desired level of control of the polymerization. Previously published compatibility tables aid in this selection,5 and many varieties of functionalized RAFT agents are commercially available. In general, there are four types of RAFT agents, all of which contain a thiocarbonylthio moiety (Scheme 1). RAFT agents such as dithioesters10 and trithiocarbonates11 work well with controlling the polymerization of “more-activated” monomers (MAMs), such as styrene (Sty), methyl acrylate (MA), and methyl methacrylate (MMA), meanwhile dithiocarbamates12,13 and xanthates14 must be used to control the polymerization of “less-activated” monomers (LAMs), such as vinyl acetate (VAc), N-vinylpyrrolidone (NVP), and N-vinylcarbazole (NVC). Improper pairing (e.g., dithiocarbamate RAFT agent with MMA monomer) will inhibit or significantly limit the polymerization.11 In an attempt to address this, there are pH-switchable RAFT agents (such as Product Nos. 751227 and 736236) capable of generating poly(MAM)-block-poly(LAM).15
RAFT agents of particular interest for drug and gene delivery allow for facile pre- or post-polymerization conjugation to biological compounds. In particular, RAFT agents containing carboxylic acid (Product Nos. 723010 and 722995), azide (Product No. 741698), NHS-ester (Product Nos. 741035 and 751227), pentafluorophenyl ester (Product No. 740810), alkyne (Product No. 765147), and thalimido end groups (Product No. 777072) and photocleavable groups (Product No. 765147) (Figure 1) are now commercially available for synthesis of well-defined homopolymers and copolymers for specific biomedical application.
Functional RAFT Polymers for Bioconjugation
Bioconjugates of vinyl polymers have been increasingly used in biomedicine, biotechnology, and nanotechnology.16 In general, they have been prepared using semitelechelic polymers having one functional end group which could be used for conjugation directly or after chemical modifications.1 While the entry of controlled radical polymerization (CRP) techniques to the bioconjugation field has enabled the in situ synthesis of bioconjugates of polymers17 as well as the direct synthesis of well-defined semitelechelic polymers suitable for conjugation to biomolecules without the need for post polymerization modifications,18,19 they have also brought the possibility of one-step synthesis of well-defined telechelic polymers. This is accomplished because the functionalized RAFT agent remains on the chain end after polymerization. Capitalizing on this inherent end group functionality, RAFT enables synthesis of various well-defined polymers for chemoselective conjugation to biomolecules. End functional polymers having carboxylic acid, azide, amine, and thiol groups have been proven to be suitable for selective bioconjugation.1 RAFT synthesized polymers with carboxylic acid end groups have been successfully bioconjugated with biotin and PEGylated biotin.20 Similarly, the amine functionalized polymer was successfully conjugated to an activated fluorescent compound, 6-[Fluorescein-5(6)-carboxamido] hexanoic acid N-hydroxysuccinimide ester (5-SFX) (Sigma Product No. 46940). It is easily imaginable that such techniques could be used to produce bioconjugates such as peptides, proteins, or targeting moieties for drug or gene delivery.
RAFT polymers containing azide and thiol were suitable for conjugation with biomolecules using “Click Chemistry.”21 Li and coworkers21adopted copper-catalyzed azide-alkyne click chemisty to synthesize responsive protein-polymer conjugates, as shown in Figure 2. In their study, BSA was functionalized with an alkyne moiety via reaction of its free cysteine residue with propargyl maleimide. Azido terminated poly(NIPAAm) was prepared via RAFT, and the protein-polymer coupling was accomplished by coppercatalyzed azide-alkyne cycloaddition (Figure 2).
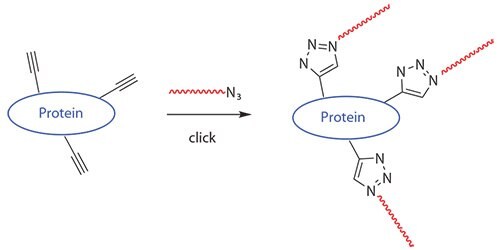
Figure 2.Polymers generated by RAFT and functionalized with a clickable azide can be used to form polymer-protein bioconjugates.
In recent years, many thiol-based reactions have been recognized and used as highly efficient processes for polymer synthesis and functionalization.22 The increasing number of routes available to transform the thiocarbonylthio end-group to a thiol provides an avenue to explore and exploit chemistry on the thiol functional ‘handle.’ Examples of reactions that can be performed at the terminal thiol group in RAFT-prepared (co)polymers include thiol–ene, thiol–yne, thiol–isocyanate, thiol–halo and thiol–oxirane reactions, many of which possess the key characteristics of click reactions.22
We developed a wide range of functional polymers with a precise molecular weight and narrow polydispersity (PDI <1.1), many of which are functionalized with carboxylic acid, azide, amine and thiol groups. These are commonly used as is or as precursors for synthesizing desired block copolymers for potential biomedical applications.
Amphiphilic Copolymers and Self-assembly
The self-assembly of amphiphilic di- and triblock copolymers into micelles and vesicles (polymersomes) has been investigated widely in pharmaceutical applications ranging from sustainedrelease technologies to gene delivery.23-26 Therapeutic molecules can be incorporated into micelles and vesicles via hydrophobic interactions, electrostatic attractions, hydrogen, and/or covalent bonds. Biodistribution, stability, solubility, immunogenicity, and nonspecific bioactivity of therapeutics can be altered using micelles/vesicles rationally designed for a particular application.20,26-27 Micellar structures can be programmed to release the therapeutics upon an environmental trigger such as temperature and pH or by passive diffusion, depending on the application.28 Immense attention in the RAFT polymerization field has been given to the generation of amphiphilic block copolymers as building blocks of micelles/vesicles for potential drug delivery applications. RAFT polymerization provides a versatile route to the generation of block copolymer micelles with controllable features, such as block lengths affecting the critical micelle concentration (thus stability), hydrodynamic size, and morphology, and chemical functionalities in the micelle corona and core offering possibilities to stabilize the supramolecular structure via covalent bonds (i.e., shell or core cross-linking), conjugating with biologically active molecules such as cell specific targeting molecules and therapeutics. We developed a series of well-defined amphiphilic polystyrene-block-poly(acrylic acid) (PS-block-PAA) copolymers using RAFT polymerization (Figure 3). The resulting copolymers show controlled molecular weight as well as narrow polydispersity indices (PDI <1.2) (Table 1). The thioester, RAFT agent end group was removed by radical induced reduction to get colorless, non-toxic polymers.
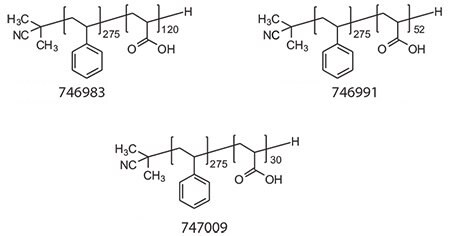
Figure 3.Structures of PS-block-PAA with PAA content 30%, 15%, and 10%, respectively.
Micellization of Polystyrene-block-poly(acrylic acid)
Micellization of block copolymers, especially those comprised of PS-block-PAA, have been investigated extensively.29 It has been found that the morphologies are influenced by many variables, including the composition of the block copolymers, molecular weight, copolymer concentration, and type of the common solvent.
Morphological overview and the molecular characteristics of PS-block-PAA are shown in Table 1. In general, micelles of ~30 nm in diameter and vesicles of 75–300 nm in diameter can be achieved by simply changing the block composition and molecular weight of the polymers.
We studied the micellization of three PS-block-PAA copolymers with PAA content of 30%, 15%, and 10% (Table 2) by dynamic light scattering (DLS).
Micelles/vesicles were prepared in 1 wt% polymer solution in 1,4-dioxane with 20% water (v/v). The DLS data show the size of the micelles/vesicles increase with the decrease of PAA content (Table 2). This has attributed due to the increase of hydrophobic content and the strong hydrophobic interaction of PS block in water. The ability to control the size of micelle/vesicle formed by using the correct block copolymer enables further research in interesting applications such as active drug loading and controlled release for biomedical application.
Conclusion
The RAFT polymers have been increasingly used in potential drug delivery applications. The potential toxicity of thiocarbonylthio groups can be eliminated easily by post polymerization treatments of the RAFT polymers.30 However, toxicity assay results determined in a few published studies31 suggest that the removal of the active thiocarbonylthio functionality from the RAFT-synthesized polymers may not always be necessary for in vitro experiments depending on the type of the RAFT agent (substituent groups), the type of the polymer and cells, and the concentration of the polymer used. While the systematic investigations on the pharmacological profile, such as metabolic cytotoxicity, of polymeric RAFT agents are yet to be performed, the increased popularity in the literature indicates that more RAFT polymers have been applied in the controlled drug delivery field by polymer chemists, material scientists, and biomedical researchers. Commercial availability of the RAFT agents and RAFT polymers will continue to enhance industrial adoption.
References
若要繼續閱讀,請登入或建立帳戶。
還沒有帳戶?