Biomedical Implant Devices Fabricated from Low Young’s Modulus Titanium Alloys Demonstrating High Mechanical Biocompatibility
Mitsuo Niinomi, Masasaki Nakai
Institute for Materials Research, Tohoku University 2-1-1, Katahira, Aoba-ku, Sendai 8577, Japan
Introduction
Biomedical implants are essentially foreign substances within the human body that must survive many years’ exposure to demanding mechanical and physiological conditions. Despite these challenges, metal implants have been widely used to substitute for or rebuild hard tissues such as bones and teeth. Metal implants are particularly useful in applications where large loads are imposed, because the mechanical reliability (e.g., fatigue strength and toughness) of metals is much higher than those of ceramics and polymers. In fact, high mechanical reliability is one of the most important properties of the metals used for implants and general structural applications. As a result, around 80% of artificial hip joints, bone plates, spinal fixation devices, and artificial dental roots are currently produced from metal.1 To achieve mechanical biocompatibility, metals used for implants must be mechanically harmonized with hard tissues. Young’s modulus is a characteristic that describes the response of a material to stress and strain that can be used to understand mechanical biocompatibility. For example, while the Young’s modulus of bone is approximately 10–30 GPa,2 that of two commonly used metals for implants, SUS 316 L stainless steel and Ti-6Al-4V ELI titanium alloy, exhibit Young’s moduli of around 200 and 110 GPa, espectively.3 As a result of this difference in Young’s modulus, the load transferred between commonly used metallic implants and bone is non-homogeneous, thereby reducing stress stimulation of the bone. This is known as the stress shielding effect. Bone is likely to atrophy under such conditions, loosening the metallic implant and frequently re-fracturing the cortical bone (hereafter, bone). Metals whose Young’s moduli are equal to that of the bone are believed to be ideal for fabricating metallic implants, and formitigating the stress shielding effect.
SUS 316 L stainless steel, Co-Cr-Mo alloys, and titanium and its alloys are the most commonly utilized metallic biomaterials used in implant devices. SUS 316 L stainless steel and Co-Cr-Mo alloys are categorized as bio-tolerant while titanium and its alloys are categorized as bio-inert. Therefore, titanium and its alloys are considered the most biocompatible of all metallic biomaterials. Futhermore, the Young’s moduli of titanium and its alloys are much smaller than other metallic biomaterials such as SUS 316 L stainless steel and Co-Cr-Mo alloys, for which the Young’s modulus is around 210 GPa.4 Titanium alloys are further categorized according to their phase constitution as α-, (α+β)-, and β-type titanium alloys. Among these alloys, the Young’s moduli of the β-type titanium alloys are much lower than those of α- and (α+β)-type titanium alloys. β-type titanium alloys are advantageous from the perspective of preventing stress shielding; therefore, researchers are developing low Young’s modulus β-type titanium alloys composed of non-toxic and nonallergenic elements for biomedical applications.
The rods used in spinal fixation devices are one example of low Young’s modulus β-type titanium alloys used in implant devices that are attracting attention. Spring-back, the degree to which a metal returns to its original shape upon release is one important characteristic of materials used in implants. The degree of spring-back in a device is affected both by the strength and by the Young’s modulus of the implanted material. While surgeons desire a higher Young’s modulus (less spring-back), patients desire a lower Young’s modulus (more spring-back). Consequently, it is necessary to satisfy the competing requirements of both surgeons and patients.5 For surgeons, the degree of spring-back in the rods should be as small as possible to minimize rod manipulability during operations. Several β-type titanium alloys having partially tunable Young’s moduli recently have been developed to address this issue. One additional consideration is a phenomenon known as “aging back”, the natural tendency for low Young’s modulus β-type titanium alloys to revert to their predominant shape after long-term use. This tendency also damages spinal fixation devices and must, therefore, be suppressed as much as possible.
This paper focuses on research to address the mechanical biocompatibility of low Young’s modulus β-type titanium alloys and reviews recent reports on this topic.
Design and Development of Low Young’s Modulus β-type Titanium Alloys
The use of non-toxic and non-allergic alloying elements is a priority when designing biologically and mechanically biocompatible titanium alloys. A variety of data can be used to select the appropriate alloying elements, including cell viability, corrosion resistance, biocompatibility, and the rate of known human allergy to various pure metals and representative metallic biomaterials.6-9 Niobium (Nb), tantalum (Ta), and zirconium (Zr) are known as the most harmless titanium alloying elements,10 while nickel (Ni) in particular, is not added due to the fact that it poses a high risk of allergic reaction. After the identification of non-toxic and nonallergic alloying elements, the reduction of the Young’s modulus remains the highest priority of materials development. There are a number of strategies in alloy design that can be used to reduce Young’s modulus. For example, large amounts of Nb and Ta, both β-stabilizing elements, are added to titanium to fabricate β-type titanium alloys. A small amount of Zr is usually added because it dissolves in both the α- and β-phases and increases the strength of the resulting alloy. The chemical composition of low-modulus Ti-Nb-Ta-Zr alloys is determined based on this concept. It is convenient to use the DV-Xα cluster-based d-electron alloy design11 because it requires a minimum number of experimental samples to determine the chemical composition of alloys. We have used d-electron alloy design to develop Ti-29Nb-13Ta-4.6Zr, referred to as TNTZ, and to conduct further research and development on this alloy. We have also developed Ti-16Nb-13Ta-4Mo, Ti-29Nb-13Ta-4Mo, Ti-29Nb-13Ta-2Sn, Ti-29Nb-13Ta-4.6Sn, Ti-29Nb-13Ta-6Sn, and Ti-29Nb-13Ta and fundamentally evaluated the mechanical biocompatibility (i.e., the Young’s modulus and mechanical properties) of the titanium alloys. Based on these analyses, we have determined that TNTZ demonstrated the best mechanical biocompatibility properties.10 Rack, et al., simultaneously developed Ti-35Nb-7Zr-5Ta.12 The Ti-36Nb-2Zr-3Ta-O alloy also has been developed for consumer applications and is expected to be used in medicine.13 The representative β-type titanium alloys used for biomedical applications are listed in Table 1.14
Due to the high cost of rare-metal elements such as Nb, Ta, Mo, and Zr, low-modulus β-type titanium alloys recently have been proposed based on using lower-cost elements such as Fe, Cr, Mn, Sn, and Al. Examples of such alloys include Ti-10Cr-Al,15 Ti-Mn,16 Ti-Mn-Fe,17 Ti-Mn-Al,18 Ti-Cr-Al,19 Ti-Sn-Cr,20 Ti-Cr-Sn-Zr,21 Ti-(Cr, Mn)-Sn,22 and Ti-12Cr.23
A number of Ni-free titanium alloys have been developed that avoid the hazardous effects posed by Ni, and many of these materials exhibit superelasticity. All β-type titanium alloys showing a single metastable β-phase and β-stability suitable for enhancing deformation-induced martensite transformation also show low Young’s moduli. They are roughly categorized into four groups:
- Ti-Nb alloys24-36 including Ti-Nb, Ti-Nb-O, Ti-Nb-Sn, Ti-Nb-Al, Ti-22Nb-(0.5-2.0)O (at%),Ti-Nb-Zr, Ti-Nb-Zr-Ta, Ti-Nb-Zr-Ta-O, Ti-Nb-Ta-Zr-N, Ti-Nb-Mo, Ti-22Nb-6Ta(at%), Ti-Nb-Au, Ti-Nb-Pt, Ti-Nb-Ta, and Ti-Nb-Pd
- Ti-Mo alloys37-41 including Ti-Mo-Ga, Ti-Mo-Ge, Ti-Mo-Sn, Ti-Mo-Ag, Ti-5Mo-(2-5)Ag (at%), Ti-5Mo-(1-3)Sn (at%), and the Ti-Sc-Mo alloys
- Ti-Ta alloys42 including Ti-50Ta, Ti-50Ta-4Sn, and Ti-50Ta-10Zr
- Ti-Cr alloys43 including Ti-7Cr-(1.5, 3.0, 4.5)Al
Other alloys such as TNTZ,44 Ti-7Cr-(1.5, 3.0, 4.5)Al super elastic and shape memory alloys, Gum Metal [Ti-25at% (Ta, Nb, V) + (Zr, Hf, O)],13 and Ti-9.7Mo-4Nb-2V-3Al45 super-elastic alloys have also been developed.
Young’s Modulus
The Young’s moduli of representative α-, (α+β)-, and β-type titanium alloys used for biomedical applications are shown in Figure 1. The Young’s moduli of the as-solutionized β-type titanium alloys lies roughly between 60 and 80 GPa, also shown in Figure 1.

Figure 1.Young’s moduli of α-, (α + β)-, and β-type titanium alloys for biomedical applications
Since the mechanical strength of as-solutionized β-type titanium alloys is generally low, their mechanical strength can be improved by introducing secondary phases such as ω- and α-phases through heat or thermo-mechanical treatments. This process, known as precipitation strengthening, increases the Young’s modulus. It has been reported that the Young’s modulus of the heat-treated or thermo-mechanically treated TNTZ increases from 60 to 100 GPa, meaning that the Young’s modulus of TNTZ tends to increase with increasing mechanical strength. It also means that TNTZ can be heat treated or thermo-mechanically treated to tune the Young’s modulus from that of the β-type titanium alloy to that of the (α+β)-type titanium alloy.
Further Reducing Young’s Modulus
Reducing Young’s Modulus by Tuning Crystal Growth Direction
It has been reported that the mechanical properties of TNTZ strongly depend on the direction of crystal growth.47 Similarly, the Young’s modulus of the β-type titanium alloy can also depend on the crystal growth direction. Severe cold-working β-type titanium alloys such as TNTZ can be used to form a texture showing preferentially aligned crystal growth. Therefore, it should be possible to grow textures consisting of preferentially oriented crystals of low Young’s modulus β-type titanium alloys such as TNTZ. Figure 248 shows the Young’s modulus of coldrolled TNTZ plotted as a function of the cold-working ratio. The Young’s modulus of the TNTZ cold-worked at 90% is around 56 GPa and that of the TNTZ not subjected to cold-working is around 65 GPa. The decrease in the Young’s modulus induced by cold-working can be attributed to texture formation. The mechanical strength (including the tensile strength and 0.2% proof stress), on the other hand, increases while the ductility (including elongation) decreases with the increasing cold-working ratio. The elongation, however, is relatively high as shown in Figure 3.48

Figure 2.Young’s modulus of TNTZ subjected to cold rolling as a function of cold working ratio.

Figure 3.Tensile properties of TNTZ subjected to cold rolling as a function of cold working ratio.
Single crystals growing in a certain direction should show a low Young’s modulus according to texture formation. Figure 449 shows the growth orientation dependence of the Young’s moduli for the TNTZ single crystals growing between the <100> and <110> directions, which were calculated by converting the cij coordinates. θ denotes the angle measured from the <100> direction on the <110> zone axis. The Young’s modulus of the TNTZ single crystals shows θ-dependent anisotropy; that is, the Young’s modulus measured in the <100> direction, E100, is approximately two times lower than that measured in the <111> direction, E111, where E100 and E111 are the lowest and highest Young’s moduli among those measured in all the directions, respectively. The lowest Young’s modulus, E100, for the TNTZ single crystal is only about 35 GPa, comparable to that for cortical bone. A Young’s modulus this low may be effective in suppressing the stress shielding in bone.
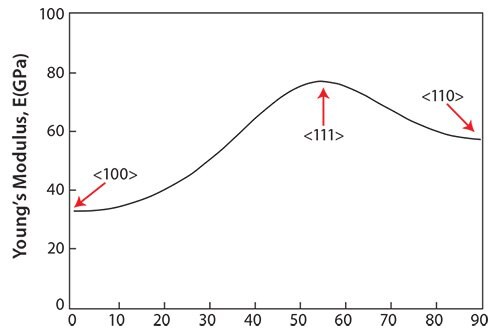
Figure 4.Young’s modulus of TNTZ single-crystal in directions between <100> and <110>.
Structurally Controlled Young’s Modulus Reduction
Introducing porosity into titanium and its alloys is a very effective method of further reducing the Young’s moduli of titanium and its alloys and it may enable the Young’s modulus to be selectively tuned. A recent report compared the relationship between the Young’s modulus and porosity of porous titanium produced from titanium powders of various diameters with those of bulk titanium.50 According to the report, the Young’s modulus of titanium with approximately 30% porosity was nearly equal to that of cortical bone. Using a titanium alloy that is less porous than bulk titanium, and whose Young’s modulus is even lower than that of bulk titanium may produce an alloy with a Young’s modulus equal to that of cortical bone. Properly sized pores also enhance bone conductivity. However, increasing titanium porosity drastically decreases titanium strength. The 0.2% proof stress of approximately 30% porosity titanium, which produces a Young’s modulus equal to that of cortical bone, is below 100 MPa. The decrease in the strength of porous titanium can be prevented by combining the titanium with a biocompatible polymer. This can be achieved by pressing high-molecular-density polyethylene (HMDP) into porous titanium, enabling the polymer to penetrate.
Another method to fabricate polymer-infiltrated titanium51 involves immersing porous titanium (pTi) into a solution containing the methyl methacrylate monomer, thereby enabling the monomer to penetrate the titanium pores. The monomer-infiltrated porous titanium is then heated to polymerize the monomer. The strength of the porous titanium increases when combined with PMMA, as shown in Figure 5.51 While the tensile strength of the PMMA-infiltrated porous titanium is greater than that of the porous titanium, the Young’s modulus of the PMMAinfiltrated porous titanium is nearly equal to that of the porous titanium (Figure 6).51 The tensile strength of the PMMA-infiltrated porous titanium reportedly further increases while the Young’s modulus remains unchanged when the PMMA-infiltrated porous titanium is treated with a silane coupling agent.

Figure 5.Tensile strength of pTi, pTi/PMMA, and Si-treated pTi/PMMA.

Figure 6.Young’s moduli of pTi, pTi/PMMA, and Si-treated pTi/PMMA.
Biodegradable poly(l-lactic acid) (PLLA) can be used instead of PMMA to infiltrate the pores of porous titanium by modifying the process used for PMMA infiltration.52 Since PLLA is biodegradable, an agent used to enhance bone conductivity could be added to the PLLA in the porous titanium in order to facilitate controlled release of the agent into the body fluid.
Balance Between Maintaining Low Young’s Modulus and Enhancing Strength or Endurance
It is important to increase strength while maintaining low Young’s moduli of titanium alloys used for biomedical applications. These two factors are contradictory because a low Young’s modulus is associated with weak bonding between atoms, while a high Young’s modulus is associated with strong bonding. The Young’s modulus of the β-phase (the main constituent of β-type titanium alloys) is generally lower than that of the α-phase (the main constituent of α- and (α+β)-titanium alloys) because the β-phase exhibits a body-centered cubic (BCC) crystal structure, where the atoms are roughly packed. The α-phase, however, has a hexagonal closed-packed (HCP) structure, with densely packed atoms. Therefore, it is more difficult to maintain the same Young’s modulus for the solutiontreated β-type titanium alloy compared to that of the as-prepared β-type titanium alloy because the strength of the as-prepared alloy can be increased through precipitation strengthening. Precipitation strengthening is used to strengthen the β-type titanium alloys while maintaining the lowest Young’s modulus possible. When considering this, balance between maintaining a low Young’s modulus and strengthening the alloy should be taken into account.
As previously shown, static strength properties such as the tensile strength of β-type titanium alloys (TNTZ) can be increased through workhardening while maintaining a low Young’s modulus. Severe-cold-working through cold rolling, swaging, or forging increases the static strength of the as-prepared β-type titanium alloy while maintaining the same Young’s modulus as that of the solution-treated β-type titanium alloy, which exhibits the lowest Young’s modulus.
Recently, severe plastic deformation has attracted much attention for its ability to increase the strength of metallic materials. Figure 753 shows the tensile properties of TNTZ subjected to high pressure torsion (HPT), a severe plastic deformation process, plotted as a function of N, the number of rotations, and those of the solution-treated and severe-cold-rolled TNTZ samples. HPT significantly increases the tensile strength, but decreases the elongation with increasing number of rotations. However, the elongation remains fairly high. The Young’s modulus of the HPT TNTZ remains almost constant, although it marginally decreases with increasing number of rotations, as shown in Figure 8.53

Figure 7.Tensile properties of TNTZ subjected to (a) solution treatment (TNTZST), (b) severe cold rolling (TNTZCR), (c) HPT at a rotation number, N, of 1 (TNTZHPT(N=1), (d) HPT at a rotation number, N, of 5 (TNTZHPT(N=5)) and (e) HPT at a rotation number, N, of 10 (TNTZHPT(N=10)).

Figure 8.Young’s moduli of TNTZCR and TNTZHPT at N= 5, 10, 40, and 60.
The dynamic strength, i.e., the fatigue strength of the severe-cold-worked TNTZ, was not higher than that of the solution-treated TNTZ.54 However, the fatigue strength considerably improved when the solution-treated TNTZ was aged or when the TNTZ was thermo-mechanically processed including severe-cold-work and aging, as shown in Figure 9.54 Aging produced the α- and ω-phase precipitates in the β-matrix. Therefore, precipitating the α- and or ω-phases can significantly improve the fatigue strength. However, it also increases the Young’s modulus because the Young’s moduli of these phases are much higher than that of the β-matrix phase (Figure 4).

Figure 9.S-N curves of TNTZ conducted with aging at 598K for 259.2ks after ST (A598K), aging at 673K for 259.2ks after ST(A673K) aging at 673K for 259.2ks after CR (B673K), and aging at 723K for 259.2ks after CR (B723K), and fatigue limit ranges of hot rolled and cast Ti-6Al-4V ELI. CR indicates cold rolling.
Precipitating the ω-phase significantly increases the strength and the Young’s modulus more than precipitating the α-phase; however, including the ω-phase also increases the brittleness of the alloy. Therefore, a small amount of ω-phase precipitate is considered optimal for improving the fatigue strength of TNTZ while maintaining a low Young’s modulus. Aging TNTZ at fairly low temperatures for a short time induces a small amount of ω-phase to precipitate and is effective for this purpose. Figure 1055 shows the Young’s modulus of the solution-treated (ST) TNTZ, severe coldrolled (CR) TNTZ, and the TNTZ aged at 573 K after solution treatment plotted as functions of aging time (AT). A tentative target for low Young’s modulus β-type titanium alloys used for biomedical applications must be between 60 and 80 GPa. The Young’s moduli of samples aged for approximately 10.8 ks remains below this target. When we evaluated fatigue properties plotted as S-N curves for ST, CR and TNTZ aged for 3.6 and 10.8 ks, we found that aging the TNTZ for 10.8 ks yields the most improvement in fatigue strength while maintaining the Young’s modulus below 80 GPa.55 Because aging the TNTZ for a short time at relatively low temperatures improves the fatigue strength while maintaining a low Young’s modulus, precipitating the proper amount of the ω-phase in TNTZ is an effective method of improving the fatigue strength.

Figure 10.Young’s moduli of ST, CR and TNTZ aged at 573K as a function of aging time: ST and CR indicate TNTZ subjected to solution treatment and severe cold rolling, respectively.
Adding a small amount of ceramic particles to the matrix is also an effective method of improving the fatigue strength of β-type titanium alloys while maintaining a low Young’s modulus. The Young’s moduli of cold-rolled TNTZ with TiB2 or cold-rolled TNTZ with Y2O3 reportedly remained nearly constant around 60 GPa with increasing B and Y concentrations while the fatigue strengths of cold-rolled TNTZ after solution treatment containing 0.1 and 0.2% B or 0.2 and 0.5% Y was improved to that of Ti-6Al-4V ELI.56
Bone Remodeling and Young’s Modulus
Animal studies were conducted on Japanese white rabbits to determine how TNTZ, SUS 316 L stainless steel, and Ti-6Al-4V ELI implants affect bone remodeling.57 Three-point bend tests were used to measure the Young’s moduli of the TNTZ, Ti-6Al-4V ELI, and SUS 316 L stainless steel bone plates and found to be 58, 108, and 161 GPa, respectively. To do this, bone plates were implanted into fracture models using rabbit tibiae. Healing conditions were observed using X-ray photographs taken at regular intervals for 48 weeks after implantation. Next, both the tibiae and the bone plates were extracted, and bone formation was externally observed. Based on X-ray images taken from 4 to 18 weeks after implantation for each plate, it was observed that the cortical bone began to thin 7 weeks after the implantation of SUS 316 L stainless steel bone plate, almost completely disappearing 12 weeks after implantation. For the Ti-6Al-4V ELI bone plate, the cortical bone first showed signs of thinning 7 weeks after the implantation, almost completely disappearing 14 weeks after implantation. For TNTZ, the cortical bone initially appeared to thin 10 weeks after implantation, almost completely disappearing after 18 weeks.57 Only the tibia implanted with the TNTZ bone plate showed an increase in the diameter of the tibia and in the double-wall structure of the intramedullary bone tissue, as shown in Figure 11.57 The inner-wall bone structure represents the original cortical bone (i.e., the remaining old cortical bone) while the outer-wall bone structure represents the newly formed bone. The bone remodeling was the direct result of using a low Young’s modulus bone plate. Therefore, using implants with a Young’s modulus that is close to that of bone can be an effective method of preventing bone resorption and is expected to lead to good bone remodeling.

Figure 11.CMR (Contact Micro-Radiograph) images showing cross sections of fracture models implanted with and without bone plates made of TNTZ at middle position (above) and distal position (below) at 48 weeks after implantation. (A) Cross section of fracture model, (B) magnification of boxed area in (C) showing branched parts of bones that form the outer and inner sides of tibiae, and (C) cross sections of unimplanted tibiae.
Low Spring-back Alloys
Spinal fixation devices are a specific type of orthopedic implant that require a low Young’s modulus to enable the formation of healthy bones.58 However, during a spinal fixation operation, a surgeon must be able to bend the device in order to reproduce the physiological spinal curvature.59 The operation is often performed within a limited space inside the patient’s body; therefore, a large amount of spring-back can be problematic. Since a lower Young’s modulus produces more springback, the Young’s moduli of the titanium alloys used for spinal fixation devices must be sufficiently low to inhibit the stress shielding effect, yet high enough to suppress spring-back.60 New titanium alloys whose predeformation Young’s moduli are low but increase during deformation (i.e., self-tuning Young’s moduli) have, therefore, been developed. Ti-Cr is the first such alloy developed and Ti-17Mo,61 Ti-30Zr-5Cr,62 Ti-30Zr-7Mo,63 and Ti-30Zr-3Mo-3Cr62 have been subsequently developed. Ti-30Zr-5Cr62 and Ti-30Zr-3Mo-3Cr62 have been simultaneously developed for use as removable titanium alloys.
Young’s moduli for a self-tunable titanium alloy, Ti-12Cr, and for solutiontreated and cold-rolled TNTZ are shown in Figure 12.5 Note that Young’s moduli of the Ti-12Cr and solution-treated TNTZ are similar (62–64 GPa). Since the cold-rolled TNTZ does not exhibit deformation-induced phase transformation, its Young’s modulus shows almost no change. However, cold rolling increases the Young’s modulus of Ti-12Cr due to the deformation-induced ω-phase transformation that occurs during cold rolling in Ti-12Cr. This is indicated in transmission electron microscopy (TEM) images.5 The formation of the ω-phase increases the Young’s moduli of β-type titanium alloys,64 which explains the increased Young’s modulus of the cold-rolled Ti-12Cr.5,65,66 The densities of cells cultured in Ti-12Cr and other alloys for 86.4 ks (24 hr) also have been reported that Ti-12Cr exhibits the highest cell density.5

Figure 12.Young’s moduli of Ti-12Cr and TNTZ subjected to solution treatment (ST) and cold rolling CR.
Decreasing the Cr content in Ti-(10–12)Cr alloys can decrease the β-(BCC)- lattice stability because Cr is a β-stabilizing element. This increases the amount of the ω-phase, which separates into the athermal and deformation-induced ω-phases formed during quenching and cold rolling stages, respectively. This suggests that lower Cr content may be beneficial for enhancing the ω-phase transformation in Ti-(10–12)Cr alloys. It is important to point out that suppressing the athermal ω-phase transformation is likely to increase the deformation-induced ω-phase transformation.67 Suppressing the formation of the athermal ω-phase in low-Cr Ti-Cr alloys should not only achieve a low pre-deformation Young’s modulus, but also increase the post-cold-rolling deformation-induced ω-phase transformation, thereby increasing the Young’s modulus.
The addition of oxygen suppresses the formation of the athermal ω-phase in titanium alloys.68, 69 For example, adding oxygen to Ti-Cr alloys increases their post-deformation Young’s moduli.70 Figure 1370 shows the Young’s moduli for solution-treated (ST) and cold-rolled (CR) Ti-(11, 12)Cr-(0.2, 0.4, 0.6)O alloys. The Young’s moduli for alloys prepared with identical Cr content increase with increasing oxygen content. This is attributed to the oxygen-induced solid-solution-strengthening of the alloys and to the ω-phase transformation. Furthermore, the Young’s moduli for Ti-11Cr-0.2O and Ti-12Cr-0.2O are almost identical, whereas Ti-12Cr-0.4O and Ti-12Cr- 0.6O show slightly higher Young’s moduli than those for Ti-11Cr-0.4O and Ti-11Cr-0.6O, respectively. This is reportedly because solution-treated Ti-11Cr-0.2O and solution-treated Ti-12Cr-0.2O still contain a small amount of the athermal ω-phase. Consequently, the competing formation of the athermal ω-phase, which can be suppressed by increasing the Cr content, stabilizes the lattice of the β-phase. This Cr-induced solid-solution strengthening of the alloys affects the Young’s modulus. Conversely, the Cr-induced solid-solution strengthening of Ti-(11,12)Cr-(0.4, 0.6)O alloys primarily determines the Young’s modulus because almost no athermal ω-phase forms; as a result, the solid solution-strengthening effect of Cr becomes the primary driver of the change in Young’s modulus.

Figure 13.Young’s moduli of Ti-(11, 12)Cr-(0.2, 0.4, 0.6)O alloys subjected to ST and CR.
The Young’s moduli for cold-rolled alloys are always higher than those for solution-treated ones. Solution-treated Ti-11Cr-0.2O exhibits a Young’s modulus below of 80 GPa, which is a much lower than those for SUS 316 L stainless steel, CP Ti, and Ti-6Al-4V ELI. Cold-rolled Ti-11Cr-0.2O exhibits a Young’s modulus above 90 GPa. This change in the Young’s modulus, which is the largest among all the materials examined, is desirable for satisfying the competing requirements of both surgeons and patients for spinal fixation applications. Figure 1470 depicts the comparative profiles for the ratio of spring-back per unit stress plotted as a function of the applied strain for TNTZ, Ti-12Cr, Ti-11Cr-0.2O, and Ti-6Al- 4V ELI. The ratio for Ti-11Cr-0.2O is always much lower than that for TNTZ, and is similar to that for Ti-6Al-4V ELI.

Figure 14.Ratio of spring-back per unit stress (R) as a function of applied strain for Ti-11Cr-0.2O, TNTZ, Ti-12Cr and Ti-6Al-4V ELI (Ti64 ELI) subjected to solution treatment.
Aging-back Characteristics
Implant rods must retain their shape over long durations. Screw-pulloutassociated post-operative correction loss is one of the most serious concerns related to spinal fixation. A number of physicians report screw pullout associated with the loss of curvature in bent implant rods over time. This behavior, known as “aging-back”, has even been observed for unloaded rods in an incubator at 310 K (37.0 °C).71 The aging-back of titanium is usually higher than that of stainless steel.72
The aging-backs of variously heat-treated TNTZ rods and of rods composed of conventional biomaterials such as pure titanium (CP-Ti), Ti-6Al-4V (Ti-64), and SUS 316 L stainless steel (SUS316L) were evaluated and are shown in Figure 15.73 The occurrence of aging-back increases exponentially with time for all the materials. Among the materials shown here, CP-Ti exhibits the worst aging-back while TNTZ-ST rod remains almost constant for over 3 months. Analysis of the temperaturedependence of these results indicates that the constituent phases contribute to the aging-back regardless of the chemical composition of the alloy. Bent rods undoubtedly age-back, and the degree of agingback depends on the type of material. The microstructures of CP-Ti, Ti-64 and TNTZ-723K, and TNTZ-ST consist of a single HCP phase, mixed BCC and HCP phases, and a single BCC phase, respectively. Analysis of the material-dependence of these results indicate that aging-back is related to the type of crystal lattice. However, further investigations are needed to elucidate the details of this relationship.

Figure 15.Aging-backs of as-solutionized TNTZ rod (TNTZ-ST), TNTZ rod subjected to aging treatment at 673K for 259.2 ks after solution treatment (TNTZ-673K) and TNTZ rod subjected to aging treatment at 723K for 259.2 ks after solution treatment (TNTZ-723K) in comparison with those of commercial pure Ti (CP-Ti), Ti-6Al-4V ELI (Ti-64) and stainless steel (SUS 316 L) as a function of time.
Summary
The results of animal tests clearly showed that the Young’s modulus of the metallic biomaterial used for implant devices to replace failed hard tissue must be similar to that of the bone to effectively prevent bone resorption and promote good bone remodeling. However, the optimal Young’s modulus required for achieving these outcomes is currently unclear and further research is necessary to determine it.
Alloy strength, ductility, and endurance must be increased while maintaining a Young’s modulus close to that of the bone; however, it is somewhat difficult to simultaneously satisfy these requirements. Establishing an alloy design or process control that enables the addition of small amounts of elements as dispersed particles should enable these requirements to be more easily satisfied in the future.
Acknowledgments
This work was supported in part by a Grant-in-Aid for Scientific Research from the Japan Society for the Promotion of Science (JSPS) and by the inter-university cooperative research program “Innovative Research for Biosis-Abiosis Intelligent Interface” of the Ministry of Education, Culture, Sports, Science, and Technology (MEXT), Japan.
References
Para seguir leyendo, inicie sesión o cree una cuenta.
¿No tiene una cuenta?