Tailoring Collagen-Based Matrices for Regenerative Medicine and Tissue Engineering
Jiayu Leong1, Liam Grover2, Hyunjoon Kong1
1Department of Chemical and Biomolecular Engineering University of Illinois, Urbana-Champaign, IL 61801 USA, 2University of Birmingham, UK
Introduction
Collagen molecules play a critical role in tissue architecture and strength, and in cell-matrix interactions as insoluble ligands to regulate the diverse phenotypic activities of cells. Whether isolated from animal tissue or produced via recombinant techniques, the enzyme-mediated biodegradability and cell adhesion properties of collagen position it as a promising building block for matrices used in both fundamental and applied bioscience. Additionally, studies have shown that collagen elicits very low immunogenicity, making it a suitable platform for the development of therapies that include long-term cell transplantation in the body, such as tissue regeneration. Efforts to further improve the performance of collagen-based materials for use in tissue engineering include the modulation of biomolecular, chemical, and mechanical characteristics using a variety of chemical conjugation and physical association techniques. For example, collagen-based materials processed in the form of gels and microporous matrices have been chemically modified to present bioactive molecules that regulate the phenotypic and secretion activities of cells of interests. In addition, the microstructure of collagen-based materials has been chemically and physically engineered to modulate its mechanical and transport properties. Through these efforts, extensive progress has been made in engineering three-dimensional (3D) collagen scaffolds into functional materials that have the potential to promote the restoration of tissues in vitro and in vivo. Here we summarize a series of recent chemical modifications and processes used to engineer a collagen-based matrix capable of improving therapeutic efficacies of regenerative medicine for the treatment of various tissue defects.
Variance of Isolated Collagen
Collagens make up a large group of triple-helical proteins that comprise the most abundant protein family found in vertebrates, representing about 30% of total proteins. In general, an individual collagen polypeptide chain has a large number of repeating amino acid sequences, most often glycine-X-Y, where X is proline and Y is hydroxyproline. Type I collagen, the most abundant in the human body, contains 31–38 lysine residues in each chain.1 Interestingly, the number and sites of lysine residues in the collagen molecule are highly conserved between human, mouse, rat, and bovine species. This small difference in amino acid composition between different species results in minimal clinical immunogenicity for medical applications, including cosmetic dermal fillers and wound healing dressings. One important source of variation among different types of collagen is the modification of lysine residues to form hydroxylysine. Hydroxylation of prolines and lysines has a significant effect on collagen fibrillogenesis and matrix mineralization.2 While the differences in hydroxylation patterns are essential in the formation of characteristic connective tissues in various parts of the body, these differences will affect the structural and chemical properties of the scaffold to be used for tissue engineering. For this reason, it important to identify the source and the chemical composition of naturally derived collagen for reliable and reproducible engineering of collagen-based materials.
Recombinant Synthesis of Collagen
To circumvent the impact of the variability of naturally derived collagen on the properties and functions of materials, recombinant techniques to manufacture homogeneous collagen are increasingly being used. Recombinant collagen molecules are produced through the transfection of mammalian cells, insect cells, yeast, or Escherichia coli with gene encoding collagen molecules. Recombinant collagens will self-assemble into ordered fibrous structures similar to that of naturally derived collagen.3 For instance, hydrogels fabricated by crosslinking recombinant human collagen type III (made in the yeast Pichia pastoris) demonstrate a comparable glucose diffusion coefficient with that of human corneal stroma.4
Recombinant techniques also have been used to introduce nonnative cysteines for bioconjugation via sulfhydryl chemistry. This is particularly useful for collagen engineering because cysteines do not naturally occur within the triple-helical region of native collagen. The fabrication of recombinant human collagen containing 2, 4, or 8 nonnative cysteines at precisely defined locations within each biopolymer has expanded the capability of collagen engineering by allowing the use of sulfhydryl chemistry to form hydrogels and immobilize bioactive factors. It is important to note collagen variants that include non-native cysteines more robustly retain their triple-helical structure and support cellular adhesion.5
Coupling Biomolecules to Collagen-based Materials
Collagen molecules have been designed to form porous scaffolds, gels, fibers, and films to direct cells in the reconstruction of damaged tissues. Additionally, collagen can be used to encapsulate and engineer cells within a matrix to elicit regenerative functions at an injured site upon transplantation. The biochemical composition and mechanical properties of the scaffold not only impact the viability and phenotype of the encapsulated cells, but also influence the function and structural characteristics of the collective cell population. The characteristics of the matrix can be tuned and customized for a specific therapeutic application by a variety of methods. For example, immobilized growth factors can be used to provide bioactive cues that enhance cellular attachment and growth of functional tissues. In addition, matrix stiffness can be controlled to instruct cells to follow different fates.
A number of chemical strategies can be used to couple collagen with molecules of interest. The primary ε-amino group of lysines on collagen is particularly reactive towards compounds containing isothiocyanates, isocyanates, N-hydroxysuccinimide esters (NHS), pentafluoroesters, aldehydes, or activated carboxyl groups. Since lingering by-products and unreacted crosslinkers can be internalized and react with other proteins found in the cells, it is important to select reagents that do not cause potential toxicities. For instance, glutaraldehyde (Product No. 3802) is an aggressive carbonyl reagent that condenses amines via Mannich reactions and/or reductive amination. However, without thorough purification, unreacted glutaraldehyde can have toxic effects because it may cause proteins in cells and tissues to crosslink.6,7
NHS esters are frequently used for the specific functionalization of biomolecules onto amino groups on collagen molecules (Scheme 1A). A wide variety of commercially produced NHS-functionalized biomolecular probes for protein labeling are now available. Conversely, aspartic and glutamic acids may be used as the anchoring groups to react with aminofunctionalized biomolecules. To improve reactivity, carbodiimides, such as 1-ethyl-3-(3-dimethylaminopropyl)carbodiimide (EDC) (Product No. 39391), are often used together with water-soluble sulfo-NHS (Product No. 56485) to activate the carboxyl groups (Scheme 1B). For instance, Hosseinkhani et al. chemically modified collagen with a lamininmimicking pentapeptide epitope consisting of isoleucine-lysine-valinealanine- valine (IKVAV). Compared with a matrix of unmodified collagen, a matrix assembled with the IKVAV-coupled collagen significantly enhances the capability of dorsal root ganglion cells to form neural networks in vitro.8 In addition, EDC/NHS ester chemistry is advantageous for coupling folded proteins to collagen while retaining the original protein conformation and bioactivity. For example, the signaling protein, vascular endothelial growth factor (VEGF), immobilized onto a collagen matrix using EDC/NHS chemistry greatly increases the viability of endothelial cells cultured in the matrix relative to VEGF in solution.9
Alternatively, Traut’s reagent, also known as 2-iminothiolane (Product No. I6256), is a small thiolation compound that reacts with primary amines to add a small spacer arm terminated by a free sulfhydryl group
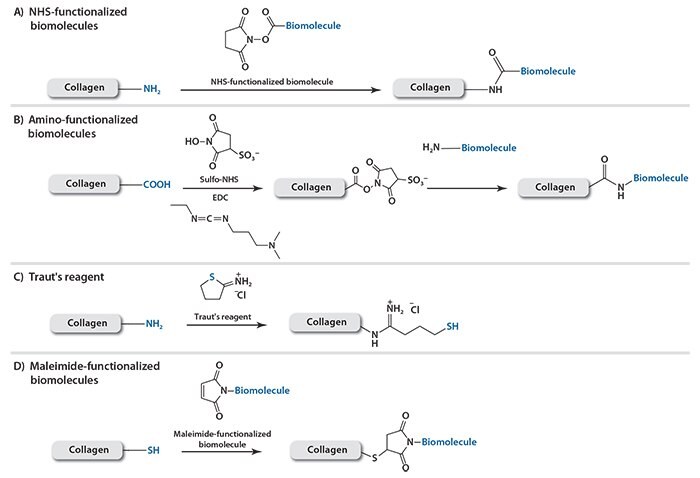
Scheme 1. Conjugation methods to functionalize collagen with A) NHS-functionalized biomolecules; B) amino-functionalized biomolecules; C) sulfhydryl group via Traut’s reagent; or D) maleimide-functionalized biomolecules.
Using this reagent, thiol-containing biomolecules can be attached to collagen through the formation of reversible disulfide bonds while maleimide-containing biomolecules can be attached via stable thioether bonds (Scheme 1C). Maleimide groups can be introduced onto peptides or proteins through sulfo-SMCC (sulfosuccinimidyl 4-(N-maleimidomethyl) cyclohexane-1-carboxylate) (Product No. M6035) (Scheme 1D). Bone morphogenetic protein-2 (BMP2) is required to induce osteogenic differentiation of host or transplanted mesenchymal stem cells for enhanced bone tissue regeneration. In order to achieve controlled release of BMP2, poly-histidine antibodies modified with sulfo-SMCC were conjugated to a collagen matrix using Traut’s reagent.10 More poly-histidine antibodies were conjugated on collagen through the chemical crosslinking than by physical adsorption, thus resulting in a collagen system that releases poly-histidine-tagged BMP2 over an extended time period. As a consequence, the engineered collagen matrix facilitated growth of viable and functional osteoblasts.
This approach was extended to simultaneously immobilize thiolcontaining biomolecules and induce crosslinking between collagen fibers in order to reduce the collagen degradation rate.11 It was demonstrated that crosslinking between internal sulfhydryl groups leads to slower degradation of the collagen matrices and moderately increases revascularization. Additionally, revascularization was further increased by immobilizing VEGF via disulfide linkages due to the sustained presence of the protein on the scaffold.
In order to gain greater spatial control, biomolecules can also be coupled to a collagen matrix in a pre-defined micropattern using benzophenone- 4-isothiocyanate and exposure to irradiation.12,13 Upon excitation with UV light, benzophenone forms a transient diradical that reacts with nearby C–H bonds from an adjacent biomolecule, forming a C–C covalent bond between the biomolecule and the matrix surface.
The aforementioned conjugation methods are limited to surface modifications because molecular diffusion prevents the penetration of the bulk material. In an effort to incorporate peptides into the bulk, Duan et al. conjugated target peptides onto amine-terminated polypropylene dendrimers.14 The remaining amine groups on the dendrimer were used as collagen crosslinkers through the chemical reaction of aspartic acids and glutamic acids in collagen (Scheme 1B), thereby incorporating the peptide into the bulk structure of the gel. Using this method, the amount of peptide can be controlled by varying the amount of peptide reacted with the dendrimer or the amount of modified dendrimer used in the collagen gel crosslinking reaction. Using YIGSR, a laminin-derived cell adhesion peptide, we showed both surface and bulk modification of collagen matrices using YIGSR peptides lead to improved adhesion and proliferation of human corneal epithelial cells as well as neurite extension from dorsal root ganglia.
Physical Association of Recombinant Proteins Fused with Collagen-binding Domains
Biomolecules also interact with each other through electrostatic and van der Waals forces. These interactions can be used in scenarios where chemical modifications are not possible or desirable, particularly for immobilizing functional proteins or cells. However, external environments can strongly affect these physical associations. For instance, biomolecules bound by electrostatic attraction may be readily influenced by increased ionic strength, decreased pH, or competing counter ions. On the other hand, interactions between charged, hydrogen-bonding, or hydrophobic groups when maximized, form strong non-covalent complexes. The avidin-biotin complex is one example of an extremely strong non-covalent association. Recombinant protein techniques have been used to incorporate specific sequences of amino acids that are able to associate strongly with their counterparts. Dai et al. found that a heptapeptide containing the TKKTLRT sequence binds stably with collagen, independent of the external environment. Upon this finding, several therapeutic proteins were engineered to associate specifically with collagen by incorporating the TKKTLRT sequence into the proteins during DNA recombination.15,16 For instance, the collagen-binding domain (CBD)- fused brain-derived neurotrophic factor (BDNF) was incorporated into collagen scaffolds; thus, sustainably stimulating cells to develop neuronal tissue such as axons.17 The CBD-fused platelet-derived growth factor-BB can also be immobilized on collagen membranes, leading to enhanced re-epithelialization of dermal ulcer wounds, deposition of the collagen, and formation of capillary lumens within the newly formed tissue area.18 Most recently, the group reported that collagen scaffolds modified with CBD-VEGF could promote urethral tissue regeneration and improve the function of the neo-urethra in a beagle extensive urethral defect model (Figure 1).19

Figure 1. Immunofluorescence staining of the normal urethra (A,B) and neo-urethra at 6 months after urethral reconstruction (C,D,E,F) with mouse monoclonal antibodies against pan-cytokeratin antibody (ab86734). The epithelium covered the total segment of neo-urethra, and the mean thickness of the epithelial layer was similar to normal urethra in the collagen-CBD-VEGF group (C,D). However, the neo-urethra was not covered by a complete epithelial layer at the central section of the neo-urethra, and fistulas were generally observed in the collagen group (E,F). The mean thickness of the epithelial layer in the collagen-CBD-VEGF group was significantly larger than that in collagen group (G).19
Besides proteins, cells can be temporarily immobilized on collagen scaffolds through an antigen-antibody association. This strategy can be used if the antibodies for cell-specific antigens are known and easily isolated. For instance, Sca-1 positive stem cells were captured in collagen gels functionalized with the anti-Sca-1 monoclonal antibody.20 When this functional collagen scaffold was transplanted into a C57/BL6 mouse as a patch to repair a surgical heart defect, enhanced regeneration of cardiomyocytes was observed.
Altering the net charge of collagen also leads to changes in the structure and association of biomolecules to collagen. Specifically, deamination, methylation, and amination have been used to modify the chemical nature of collagen prior to fabrication of collagen-glycosaminoglycan (GAG) co-precipitate.21 Deamination replaces the positive charges of the protonated ε-amino group of lysine and hydroxylysine with a neutral hydroxyl group. On the other hand, carboxyl groups of aspartic acid and glutamic acid residues in the collagen can be modified via methylation and amination. Methylation esterifies the carboxyl groups which, in turn, raise the isoelectric point. Conversely, amination converts the negatively charged carboxyl groups to positively charged amino groups and increases the cationic charge density of collagen. Since GAGs are negatively charged, the amount of GAGs retained after 6 days was found to be the highest in aminated collagen (60%) followed by methylated collagen (40%). In contrast, most GAGs were lost in the untreated group and the deaminated group within a day.
Additionally, the net charge of collagen influences intermolecular selfassembly and fibrous morphology of the matrix.21 Deaminated collagen forms thick fibers with large mesh size and few granules, while aminated collagen forms thin fibers with small mesh size and abundant granules, similar to the ultrastructure of the matrix in native rabbit nucleus pulposus. While the three chemically modified collagen scaffolds are equally able to support survival of human mesenchymal stem cells (MSCs), cells grown on aminated collagen have been shown to express little integrin αv. It is speculated that the interactions between GAGs and aminated collagen might mask the ECM binding sites for integrin αv.
These examples indicate that the sustained presence of biochemical signals has significant effect on cellular behavior. Still, another major aspect to forming effective tissue engineering scaffolds is the mechanical properties of the collagen matrices.
Tuning Mechanical Properties of Collagen Matrices
Efforts have also been made to modulate the mechanical stiffness of collagen-based materials in order to retain structural integrity and further regulate the biological activity of cells transplanted for tissue regeneration. In the past, the amount of crosslinking between collagen molecules was typically altered using cytotoxic cyanuric chloride (Product No. C95501).22 Now, the elastic modulus of a collagen gel can be controlled under non-toxic conditions using a series of aminereactive PEG derivatives such as poly(ethylene glycol) di-(succinic acid N-hydroxysuccinimidyl ester) (Product No. 713783). Varying the amount of PEG in collagen-based hydrogels can be used to tune the stiffness of the collagen gel.23,24 The different collagen gel stiffness affects adhesion and phenotype of cells loaded in a collagen gel (Figure 2).
Non-reactive PEG chains incorporated in a collagen gel can be used to modulate mechanical stiffness and the diameter of individual collagen fibers by increasing the number of bound water molecules.25 In particular, collagen gels become softer with increasing concentrations of PEG. The diameter of collagen fibers also increases with the concentration of PEG chains, thus recapitulating large perimysial collagen cables. Fibroblast cells cultured in the collagen-PEG gel have been shown to be responsive to the diameter of collagen fibers and form large cell bundles with extensive cell–cell contact, characteristic of the contractile proto-myofibroblast found in wound healing.
Another approach to tuning collagen gel stiffness is to introduce interpenetrating networks (IPN) using polymers that present methacrylate groups. For instance, collagen-containing poloxamine hydrogels were prepared using a four-arm PEO-PPO block copolymer (poloxamine, Tetronic®) in order to create gels that present methacrylate groups. The free radical crosslinking reaction of the polymer within pre-formed collagen gels leads to a sharp increase in stiffness compared to pure collagen gels.26 Another example of an IPN gel is a network of collagen fibers crosslinked with polyacrylamide (PAAm) (Prod. No. 749222) networks. This gel is prepared by inducing a Michael reaction between the amine groups of collagen and the vinyl groups of acrylamide. The resulting collagen fibers are chemically connected to the PAAm gel

Figure 2. Effects of hydrogel elastic modulus E0 on proliferation of 3D hepatocarcinoma spheroids. A,B) Spheroids formed within the pure collagen gel (E0 = 9.7 kPa; 1st row) were larger and more disorganized than those formed within the collagen-PEG gels (E0 = 4.0 kPa; 2nd row). Intracellular actins were stained with phalloidin (green), and the nuclei were stained with DAPI (red). Images in B) are magnified views of spheroids shown in A). C) Spheroids grown in the pure collagen gel displayed higher integrin expression (yellow) than those grown in the collagen-PEG gel. Cell nuclei were stained with DAPI (red). D) Spheroids grown in the collagen-PEG gel expressed higher levels of E-cadherin (blue) compared to those grown in the pure collagen gel. Cell nuclei were stained with DAPI (red). Scale bars represent 50 μm.23
Without interfering with the crosslinking reaction between PAAm, one interesting observation is that the resulting IPN gel system enables control of the elastic modulus without significantly altering the degree of gel swelling.27 The advantage of the decoupled control in these two parameters is that the gel loaded with cells or drug molecules is able to retain its structural integrity and at the same time is permeable to biomolecular transport. This is unlike other gels where there is typically an inverse dependence between water permeability and stiffness. As a result, in a sufficiently rigid matrix there is a compromise between cell viability and therapeutic availability.
Conversely, semi-IPNs (in which only collagen is three-dimensionally associated via physical crosslinking and secondary polymer chains are entangled with the collagen gel network) form less dense gels with lower storage modulus and higher degradation rate. With these differences in properties, zonal distribution of crosslinking density, viscoelasticity, water content, and pore sizes at micro- and macroscales matricies can be constructed by patterning the hydrogel with IPNs and semi-IPNs.28 These controlled differential structural modifications within the same scaffold are useful for regenerative medicine, which involves co-culturing of more than one type of cells, each requiring matrices with different mechanical properties.
The final approach to regulating the mechanical properties of the collagen gel is through introducing microparticles into collagen gels (Figure 3). For example, incorporating hydrolyzable poly(lactic-co-glycolic acid) (PLGA) microparticles (see product table on page 111) within the collagen gels can increase both the gel’s elasticity and rigidity due to the association of PLGA microparticles with the collagen fibrils.29 Interestingly, this approach substantially increases the elastic modulus of the gel without altering the biomolecular diffusivity within the gel. In addition, the PLGA microparticles significantly enhance the deposition of apatitelike minerals within the gels when incubated in simulated body fluid or encapsulated with mesenchymal stem cells. The favorable osteogenic conditions contributed by the microparticles result in enhanced formation of bone-like tissues.

Figure 3. Analysis of minerals formed within MSC-encapsulating hydrogels. A,B) SEM images of minerals formed in the MSC-encapsulating collagen (A) and PLGA-Col (B) hydrogels after 0, 5, and 10 days of incubation in osteogenic differentiation medium. C) EDS spectra of minerals formed within the gels. PLGA-Col hydrogels contained 10 mg/mL PLGA particles, while pure collagen gels contained 0 mg/mL.29
Summary
This article presents a toolbox of strategies and techniques to customize collagen gels with desired biochemical, mechanical, and transport properties. The highlighted studies serve to greatly advance controllability of structure-property-function of collagen-based materials used for both fundamental and applied bioscience studies relevant to regenerative biology and medicine. Additionally, the underlying principle of material design will be useful in assembling a wide array of biomaterials used for cell culture, drug delivery, and therapies.
References
계속 읽으시려면 로그인하거나 계정을 생성하세요.
계정이 없으십니까?