Metal Hydrides for NiMH Battery Applications
Dhanesh Chandra*, Wen-Ming Chien, Anjali Talekar
University of Nevada, Reno, College of Engineering Reno, NV 89557
Material Matters Volume 6 Article 2
Introduction
Rechargeable solid-state batteries are becoming increasingly important due to wide-spread use in computers, portable electronics, and vehicular applications. The Partnership for a New Generation of Vehicles (PNGV), a collaboration between the U.S. government and auto industry, was initiated in 1996 to promote the development of hybrid electric vehicles (HEVs) with significantly increased fuel economies. As shown in Figure 1, metal hydride and Li-ion batteries have high energy densities and are the most promising classes of modern rechargeable batteries.1 Li-ion batteries are very attractive for modern portable electronic devices, and nickel metal hydride (NiMH) batteries are a significant component of modern hybrid automobiles. Based on 1996 prices, the estimated cost of these materials was <$1/gH produced (Table 2).2-4 Although both NiMH and Li ion batteries are equally important for various applications, the cradle-to-gate (ctg) life Ectg/kg basis is somewhat higher than that of other batteries (Figure 2).5 This article is a summary of rare earth intermetallic compounds and their structures, properties, technologies, and applications, particularly with regard to rechargeable batteries.
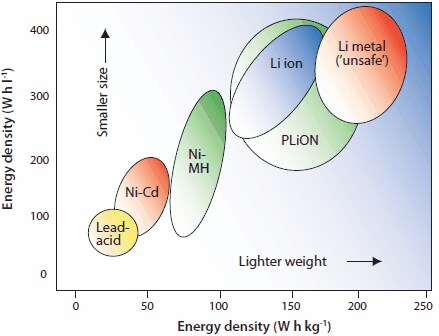
Figure 1.Comparison of volumetric and gravimetric energy density of the important NiMH, lead-acid (PbA), Ni-Cd, Na/S, and Li–ion batteries.
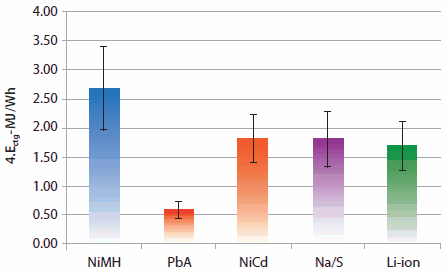
Figure 2.Average cradle-to-gate life (Ectg) per watt hour basis with one standard deviation for NiMH, lead-acid (PbA), Ni-Cd, Na/S, and Li–ion batteries.
Metal hydrides have been widely investigated over the years, with significant interest developing in the 1960s. The majority of research activities prior to 1960 are reported in Metal Hydrides, a book by Mueller, Blackledge, and Libowitz.6 More recent work is covered in several good reviews by Schlapbach,7 Sandrock,8 Yvon,9 Fukai,10 Walker,11 and others. In general, metastable binary or higher-order alloys that reversibly absorb/desorb hydrogen are preferred in applications due to their metastable nature: pure elements tend to form comparably stable hydrides. The precursor alloys that are used for hydrides are broadly classified into six categories based on A-B component systems. Examples of simple binary systems, with no substitutions, are:
- AB (HfNi, FeTi)
- AB2 (Mn2Zn, TiFe2)
- A2B (Hf2Fe, Mg2Ni)
- A2B7 (Pr2Ni7, Ce2Co7)
- AB3 (NdCo3, GdFe3)
- AB5 (LaNi5, CeNi5)
The AB5-type, LaNi5H6.7 hydrides have high volumetric capacities (~130 kgH2/m3), low gravimetric capacities (~2 wt.% H2), and operate near room temperature. To help understand the development of these hydrides, the unsubstituted A-B hydrides will be discussed first in terms of hydrogen capacities. The following discussion will focus on the effect of one component substitution in the A-B systems. Finally, this article will address more practical hydrides, some of which are commercialized.
Thermodynamics and Crystal Structures of NiMH Battery Materials
Thermodynamics
Metal hydrides were first used for storage of hydrogen in the solid state. The alloys used for metal hydrides in NiMH battery applications are mainly AB5- and AB2-type; other alloy structures may be under consideration but will not be addressed here. Table 1 describes some important properties of these model structure types.
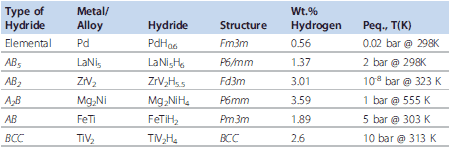
Table 1.Intermetallic Compounds and their Hydrogen Storage Properties.
The most important properties for battery applications are the hydrogen capacity, isotherm pressure, hysteresis, volumetric expansion/contraction, enthalpies, and hydriding/dehydriding temperatures. Additional properties are important for practical applications. These include the activation of alloys, decrepitation, kinetics, heat transfer, gaseous impurities in H2, cyclic ability, safety, metallurgical fabrication of the alloys in large scale, cost, and recycling.
Thermodynamic properties are obtained by measuring volumetric isotherms using a Sieverts apparatus; these are now commercially available. Typically, we obtain isotherms at a constant temperature, yielding the H/M ratio and wt.% H. As the alloy starts to absorb hydrogen, a solid solution region is observed, called the α-phase. As more hydrogen is absorbed, the α→β phase transition occurs, giving the hydride phase in the plateau region. A schematic of this is shown in Figure 3; the x- and y-axes represent the hydrogen/metal ratio and pressure, respectively. Next, we measure many isotherms at different temperatures and obtain mid-plateau pressures. One generally observes hysteresis in the isotherms between desorption/absorption. Plotting ln P vs. 1/T(K) yields the van′t Hoff plot, and the slope of the van′t Hoff line gives ΔH/R. The enthalpy of formation is given by (-ΔH/R). The desired properties of the hydrides are estimated by creating a window of operation, f(p,T). van′t Hoff plots for many of the important hydrides are shown in Figure 4; these will be discussed later in the following sections. The α→β phase transition for AB5 materials is described by the equations given below:
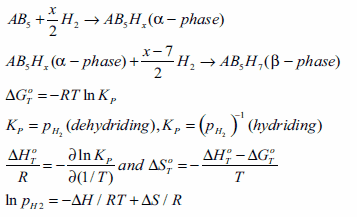
Here, M = metal or alloy (ex: LaNi5), which first forms a solid solution phase with hydrogen, MHy, designated as the α-phase. Then, this α-phase forms a hydride, MHx. In the case of LaNi5 (Prod. No. 685933) , the LaNi5-H phase forms first and then the LaNi5H7 forms. There is only a solid solution region beyond a certain temperature (Tcritical). The most important metal hydrides include several rare earth materials, which are listed in Table 1.3, 4
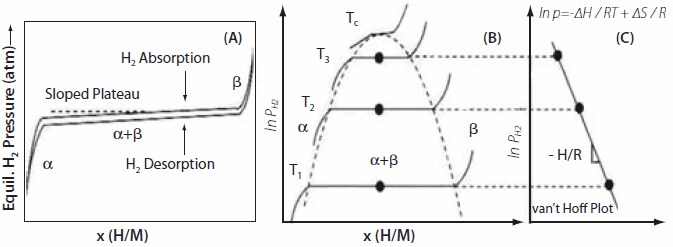
Figure 3.(A) An isotherm showing a sloped plateau for hydrogen absorption and desorption isotherms. Hysteresis between absorption and desorption isotherms is also shown. (B) The effect of temperature on the isotherm plateau pressure and phase transitions regions from α → α + β → β are shown. (C) The van’t Hoff plot derived from the isotherms obtained at various temperatures; whose slope yields the enthalpy of hydriding.
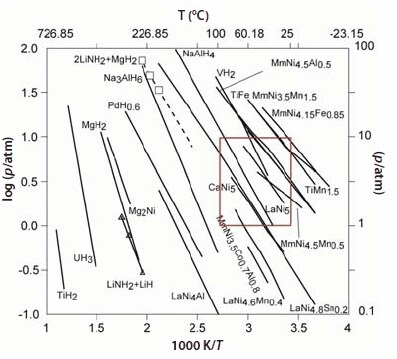
Figure 4.A compilation of the van’t Hoff plots of selected elemental, classical, and complex hydrides. The boxed area represents the desired temperature and pressure range of operation for vehicular applications.
As will be discussed later, there are many substituted compounds that effect thermodynamic properties. Some simple examples of cycling effects from our work on substituted LaNi5 show significant disproportionation after 10,000 cycles (~1 h each) suggesting loss of hydriding properties (Figure 5). Simple Ni-site substitutions with small amounts of Sn improved cycle life significantly; even after 10,000 cycles there is no significant loss in hydrogen capacity in LaNi4.8Sn0.2 (Figure 6). Further details on thermal cycling and aging of substituted LaNi5 alloys can be found in Lambert et al.,15 Chandra et al.,16 and Percheron-Guegan et al.17 The knowledge of long-term cycling and thermal aging behavior of hydrides is essential to evaluate the hydrogen storage performance over a period of time. Thus, proper substitution of A or B in AB5 or AB2 can enhance cycle life, corrosion resistance properties, hysteresis, and electrochemical properties. Optimal stoichiometry battery alloys will be discussed in the following sections.
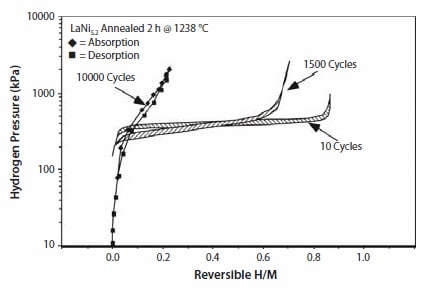
Figure 5.Isotherm of LaNi5.2 taken at 25 °C after intrinsic P-T cycling for 10 (activation), 1500, and 10,000 times, showing severe degradation of this hydride.15
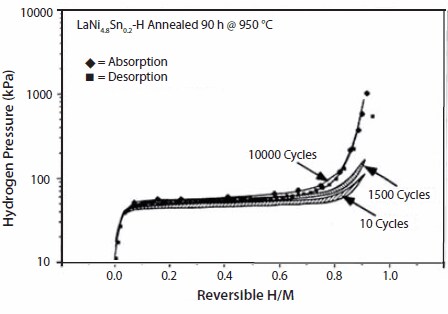
Figure 6.Isotherms of LaNi0.8Sn0.2 taken at 25 °C, before and after P-T intrinsic cycling for 1500 and 10,000 times showing virtually no loss in hydrogen capacity in the case of LaNi0.8Sn0.2.15
Crystal Structures of Battery Materials
Important properties for the MH battery are durability/cycle life, hydrogen capacity, and charge/discharge efficiency. From this standpoint, rare earth AB5 and AB2 metal hydrides are most desirable for longrange use in plug-in-hybrid-electric vehicle (PHEV) and electric vehicle (EV) applications. These are referred to as NiMH batteries and operate around ambient temperature. For example, the Toyota™ Prius (II-V models) use sealed NiMH batteries, which are estimated to have a 150,000 mile battery life based on the manufacturer′s laboratory bench testing.19 With further developments in the cycle life and energy densities of NiMH batteries, the upcoming electric vehicles may use these reliable high energy density batteries. These types of hydrides are now called “Classical Hydrides,” but they have remarkable battery properties that are achieved by micro-alloying both A and B components, increasing the electrochemical and cycle life of the batteries.
Crystal Structure of AB5 and AB2 Hydride Materials
The crystal structures for the AB5 and AB2 compounds are listed in Table 2. The AB5 compounds are line intermetallic compounds. AB2 are intermetallic or intermediate compounds (with a small homogeneity range of compositions) and may not have the exact 1:2 stoichiometric composition.
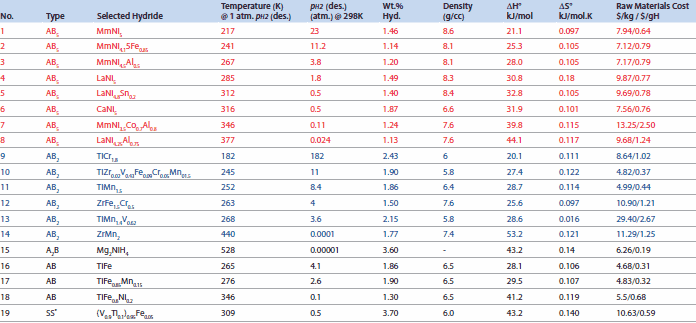
Table 2.Selected classical hydrides with their hydrogen capacities and plateau pressures (Prices basis: mid-1996).3, 4, 8
Structure of AB5 Compounds
Many NiMH batteries are made out of AB5-type structure materials. The main base alloy materials are LaNi5 and MmNi5 (mischmetal-nickel alloy, Product No. 685976), and the A and B components are invariably substituted in these materials to improve properties. The model compound, LaNi5, has a hexagonal crystal structure, Strukturbericht D2d (CaCu5-type, P6/mmm, a = 5.0228 Å, and c = 3.9826 Å). In the LaNi5 structure, there is one site for La (1a site) and two different sites for Ni. The first Ni-site (2c site) is surrounded by La atoms and the other Ni-site (3g site) is located in the middle layer of the hexagonal structure with only Ni atoms. In many cases there is excess Ni (e.g., LaNi5.2) and this varies the c/a ratio.20 The structures of both the intermetallic LaNi5 and the hydride are shown in Figure 7.20-22
.gif)
Figure 7.Crystal structure LaNi5 20, 21 and LaNi5H7.22
The hydride is LaNi5H7, which is generally ordered and is a line compound. Latriuge reported the structure to be a hexagonal double cell with a space group P63mc, a = 5.409 Å, and c = 8.6 Å.21, 22 Joubert et al.,23 Thompson, Reilly and Hasting,24 and many other groups have also reported this structure.
Practical battery materials are based on the AB5 model. In one of the optimum commercial alloys, the A-site of LaNi5 is substituted with mischmetal (Mm), which is a mixture of many elements ranging from atomic number 51 to 71. The B-site is substituted with Co-Al-Mn. Manufacturers of batteries have their variation of substitutions that are proprietary, but some promising alloys are listed in Table 2 (Nos. 1 through 8). The alloy MmNi3.5Co0.7Al0.8 (shown in Figure 4 and Table 2) appears to be appropriate for battery properties and has the highest raw materials cost normalized to reversible capacity. Due to cost and other considerations, Co is not very desirable but is alloyed in small quantities for corrosion resistance.
Structure of AB2 Compounds
Materials of the AB2-type are generally Laves phase structures (Strukturbericht C14 and C15). This is another class of NiMH batteries; some important materials are listed in Table 2 (Nos. 8 through 14). These materials also charge/discharge at near ambient temperatures like the AB5 hydrides. The C14 materials may have cubic, orthorhombic, or hexagonal structures. Figure 8 shows two types of common structures for C14 and C15 phases. An important example of C14 is ZrMn2, which has a hexagonal structure with Z = 4 (ZrMn2/formula unit) in the unit cell and is used for gas separation applications. The C15 structure of AB2 has Z = 8/formula unit in the unit cell, as seen in ZrV2-type materials; Yvon reported that H atoms occupy the A2B2 tetrahedral interstices.27 Examples of AB2 compounds include GdMn2, HfV2, CeNi2, and TiCr1.8.
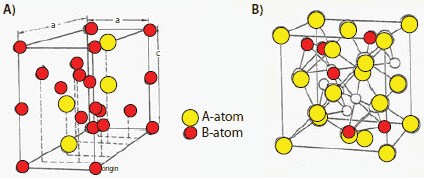
Figure 8.(A) One of the structures of the C14 Laves phases25 and (B) one of the structures of the C15 Laves phases.26
In this AB2 case, the common elements for A are Ti, Zr, Hf, and other rare earths (excluding Lu). Common B-site elements are Cr, V, Fe, and Mn. Many substitutions may be made for A and B to improve battery performance. Daimler Benz developed TiZr0.02V0.43Fe0.09Cr0.05Mn01.5 (Table 2, No. 10, Product No. 685941), a commercial alloy that has a reasonable price.28
The third class of materials that may have potential in NiMH batteries are AB hydrides. These generally have "CsCl"-type structures (Strukturbericht B2, space group Pm-3m). Libowitz29 first reported ZrNi hydrides that hydrided around 100 °C, and in 1974, Reilly30 developed an FeTi alloy (Table 2, No. 17) that formed FeTiH and FeTiH1.5 and exhibited two plateaus at 30 °C. Reilly31 also discovered TiFe0.7Mn0.2, which shows an H/M = 1 at 40 °C, and, Sandrock32 developed TiFe1-y Aly (y = 0.04 to 0.10), TiFe0.85Mn0.15, and TiFe0.8Ni0.2 (Table 2, Nos.17 and 18).32
Substitutions of A and B in AB5 and AB2 Compounds
The line compound hydrides are LaNi5, YNi5 (Product No. 693928) , CeNi5, MmNi5, and others. The A and B components are generally substituted to yield the most desirable properties. For example, the isotherm properties are modified to give a desirable plateau pressure, in some cases nearly two orders of magnitude near operating temperature. The LaNi5 is a model compound that has a plateau pressure of 1.5 atm; NdNi5, CeNi5, and others also have high plateau pressures. A-site substitutions involve commercial mischmetal alloy mixtures (La, Pr, Ce, Nd). In this case hysteresis is increased but the hydrogen storage capacity is not reduced. Partial substitutions are also possible, in compounds such as Mm1-xCaNi5, which yield reduced plateau pressures as well as hysteresis. B-site substitutions of Ni with Pt, Cu, and others have not led to useful compounds. Partial substitution of the B-site, on the other hand, has led to many changes in thermodynamic properties. For battery applications, it is important that there is minimum volume change during hydriding/dehydriding; it was shown that addition of Co reduces volumetric change. Sakai reported that fully substituted MmNi5, used in battery electrode applications, has some Co content to improve electrode life.37 There are other patents and citations that have not been included in this review for brevity. The commercial B-substituted MmNi5 alloys containing Co, Al, and Mn exhibit plateau pressures below 1 bar and good corrosion resistance. It is suggested that the commercial electrode alloy composition is approximately MmNi3.5Co0.8Mn0.4Al0.3. This alloy has great commercial value for battery applications as well as other hydrogen storage alloys for fuel cell and other stationary hydrogen storage applications. Partial B-site substitutions with Sn, Mn, In, and Si have also yielded superior performing hydrides. The most interesting ones that produced the optimum hydriding properties are Al and Sn. The well-known LaNi4.5Al0.5 has been used for Tritum applications. An example of single B-element substitution is shown for LaNi5.2 and LaNi4.8Sn0.2 (Figures 5 and 6). It can be seen that thermal cycle life is profoundly affected by changes in alloying element. Bowman improved the properties of these alloys, which have been used for heat pumps in space applications.33 In addition, it was found that cycle life improvements are possible with these substitutions.34 Rare earths are also substituted in AB2 compounds; compounds such as MmMnAl, LaMnAl, TiZr0.02V0.43Fe0.09Cr0.05Mn01.5, and TiMn1.4V0.62 are equally important for our applications. Sandrock and Goodell also performed ambient pressure cycling on Fe0.85Mn0.15Ti0.5 and showed the effect of gas impurities upon cycling.35
Electrochemical Reactions in NiMH Batteries
A schematic of a NiMH battery is shown in Figure 9, where the metal hydride is the negative electrode, NiO(OH)/Ni(OH)2 is the positive electrode, and KOH is the electrolyte. On the positive side, the Ni(OH)2 electrode oxidizes to NiO(OH) during charging and reduces back to Ni(OH)2 during discharging. At the negative electrode, the metal hydride reduces to the alloy during discharge. The charge-discharge redox equations are also shown in Figure 9. It should be noted that there is no precipitation mechanism in this type of battery, as seen in Ni/Cd and others; the charge/discharge mechanism proceeds via proton transfer between Ni hydroxide and the metal hydride.
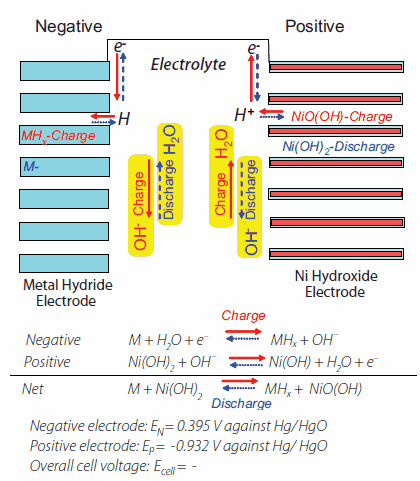
Figure 9.Charge-discharge mechanism in a NiMH battery.
Over the years, these AB5 compounds have been optimized for long cycle life, structural integrity, corrosion resistance, and low cost. It should be noted that substitution of AB5 or AB2 hydrides has a profound effect on the hydride properties, which have been optimized by battery manufacturers. The decrepitation of AB5 electrodes was improved by addition of Co, Mn, and Al to LaNi5 (Product No. 685968) . Koriyama reported the electrochemical durability of the electrodes due to substitution of various elements in LaNi5 electrode, and these are listed in Table 3.36
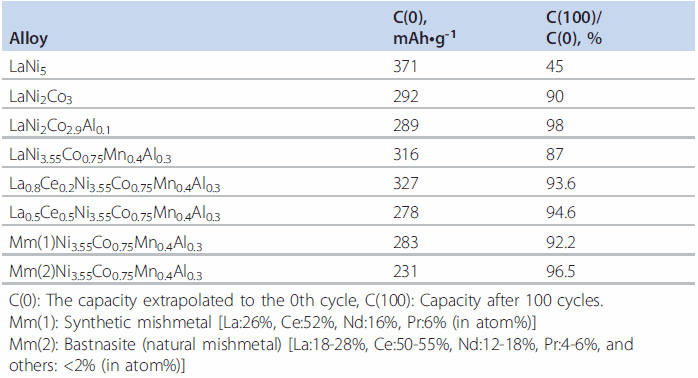
Table 3.Effects of substitution of La in LaNi5-based alloy with other rare earths.
Typically, Mm(Ni,Co,Mn,Al)5 powders or closely related compositions are used for batteries. The driving range for Mitsubishi® Libero EV has been established at 220 miles at 28 kwH battery storage energy based on 1999 report.3 The cycle life of NiMH batteries is generally >1,000 with (practical) specific charge of 70-80 Wh/kg, although the theoretical charges are in the range of 220 and 230 Wh/kg for AB5 and AB2, respectively.37 For the MmNi3.6Al0.4Co0.7Mn0.3 alloy, the discharge capacities of the alloy remain at 250 mAh/g with a nominal fading in capacity (to the extent of ~20 mAh/g) after prolonged cycling. Studies have also been performed on complex substituted alloys (from Sweden) of MmNi5 [(La0.58Ce0.29Nd0.08Pr0.05) (Ni3.6Co0.7Mn0.3Al0.4)] that showed improved activation and a high rate of discharge capacity by using sintered hydride electrodes.38 Another example of cycle life of LaNi4.7Al0.0.3 as compared to Mm0.95Ti0.05Co0.45Mn0.35Al0.15 is shown in Figure 10.39 Another example of electrochemical cycling of LaNi4.8Sn0.1+x showed that LaNi4.8Sn0.2 had better cyclic stability than the off-stoichiometric alloys such as LaNi4.8Sn0.1 and LaNi4.8Sn0.4 (Figure 11).

Figure 10.Discharge capacity of Mm0.95Ti0.05Co0.45Mn0.35Al0.35 and LaNi4.7iAl0.3.
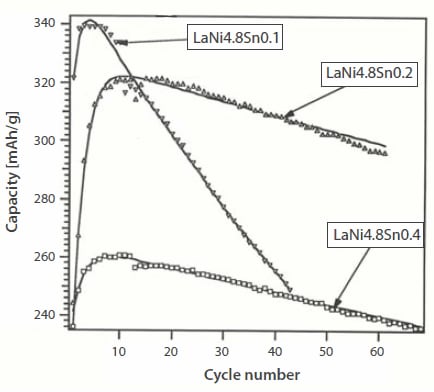
Figure 11.Discharge capacity of off-stoichiometric alloy, LaNi4.8iSn0.1+x.
Societal Impacts of the Use of NiMH Batteries
NiMH hydride batteries are used in hybrid automobile batteries, electric razors, toothbrushes, cameras, camcorders, mobile phones, pagers, medical instruments/equipment, and numerous other applications. Because Li-ion battery technology is developing at an accelerated rate, development of NiMH battery materials must continue in order for this technology to remain competitive. NiMH batteries, however, appear to have slightly better life cycles than Li-ion batteries (Figure 2) on a MJ/Wh basis. It should be noted that the 2010 Toyota™ Prius uses Sanyo® Electric Co. NiMH batteries.
Metal hydrides have several other applications besides battery technology. Among them is hydrogen storage for vehicular fuel cell and internal combustion (IC) engine applications. These have great environmental implications, as the hydrogen vehicles emit water rather than CO2. Many companies have made prototype hydrogen/fuel cell operated automobiles; Frank Lynch at HCI in Colorado developed metal tanks in the 1970s for the operation of Caterpillar® tractors using these hydrides. The main issue for practical modern automobiles is the development of reliable light-weight hydrides that are reversible and have approximately 10 wt.% H storage capacity. With additional development and optimization, it is hoped that some of the battery alloys listed in Table 2 may someday be used for this purpose.
References
To continue reading please sign in or create an account.
Don't Have An Account?