Electrospun Nanofibers for Drug Delivery Systems
Sang Jin Lee, James J. Yoo, Anthony Atala
1Wake Forest Institute for Regenerative Medicine, 2Wake Forest School of Medicine Medical Center Boulevard, 3Winston-Salem, NC 27157, USA
Introduction
Local delivery of bioactive molecules using an implantable device can decrease the amount of drug dose required as well as non-target site toxicities compared to oral or systemic drug administration.1-3 Recently, electrospinning techniques using high-voltage electrostatic fields to generate nanofibrous structures have been used to develop drug or protein-loaded electrospun nanofibrous constructs for use as a novel implantable delivery system. The biomimetic cellular environment provided by electrospinning technology resembles the extracellular matrix (ECM) of native tissues and allows for the controlled fabrication of nano- to micro-scale fibers with specific composition, structure, and biomechanical properties of the fibrous constructs.4-8 Recent studies have shown that electrospun fibers with inherently high surface-areato-volume ratio and high interconnectivity have a number of benefits, including: (1) high drug loading efficiency; (2) the ability to overcome mass transfer limitations associated with most polymeric delivery systems; (3) the facilitation of drug diffusion; and (4) improvement of solubility of various bioactive molecules.9 Encapsulation of these bioactive molecules into electrospun fibers allows for localized delivery of antimicrobials, anti-inflammatories, antiscarring agents, antineoplastic agents, immunosuppressives, growth factors, cytokines, genes (DNA and RNA), enzymes, bacteria and viruses, and a number of other important bioactive ingredients to target sites.
Electrospinning Apparatus
Electrospinning has been used extensively to synthesize nanofibers for various applications4,6,10-12 because it allows the precise control over the composition, structure, and mechanical properties of the resulting biomaterial. Electrospinning requires a high-voltage power supply, a syringe pump, a polymer solution to be spun, and a grounded collection surface. Polymer solutions are exposed to a high-voltage power supply and delivered with a syringe through a blunt-tip needle using a syringe pump. Nanofibers can be collected onto a specially designed grounded mandrel placed at a specific distance from the needle tip. Controlling certain variables such as polymer solution concentration, molecular weight, conductivity, surface tension, flow rate, distance, collector geometry, and rotation speed during electrospinning allows for the fabrication of fibers with a specific morphology, diameter, and alignment. The fabricated electrospun nanofibers possess unique features and properties, including an extremely high surface-area-to-volume ratio that enhances cellular interactions with their substrates. The process of electrospinning also allows localized delivery of a combination of bioactive molecules to cells over a prolonged period.10 Conservation of the structural integrity and bioactivity of the encapsulated drug or protein after the electrospinning process is critical; therefore, several modifications of the electrospinning apparatus are currently being explored to better enable the fabrication of improved drug delivery systems (Figure 1).3
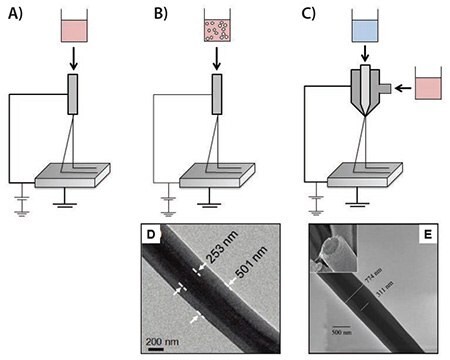
Figure 1.Illustrations of different electrospinning techniques for drug/protein delivery. A) Blend electrospinning, B) Emulsion electrospinning, C) Coaxial electrospinning, D) Core-shell structure nanofibers comprised of a poly(vinyl pyrrolidone) (PVP) shell and a polycarbosilane (PCS) core prepared by emulsion electrospinning,13 and E) Core-shell structure nanofibers composed of a poly(ε-caprolactone) (PCL) shell and a bovine serum albumin (BSA)-loaded poly(ethylene glycol) (PEG) core prepared by coaxial electrospinning.14
Modulation of Controlled Release from Electrospun Nanofibers
Since the release of bioactive molecules occurs primarily by diffusion, it has been demonstrated that the release profile of bioactive molecules from electrospun fibers can be influenced by biodegradability, fiber diameters, hydrophilicity, hydrophobicity, and configuration.10 In a recent study conducted by our group, electrospun PCL/collagen type I scaffolds derived from calf skin and composed of various fiber diameters were fabricated under conditions in which the concentration of the solution (Figure 2), flow rate, needle diameter, and distance from the substrate were carefully controlled.6 The fabricated electrospun PCL/collagen scaffolds showed randomly oriented fibrous structures with a linear relationship between fiber diameter and solution concentration. Distance between dispenser tip, collector, and needle gauge also affected the fiber diameters.
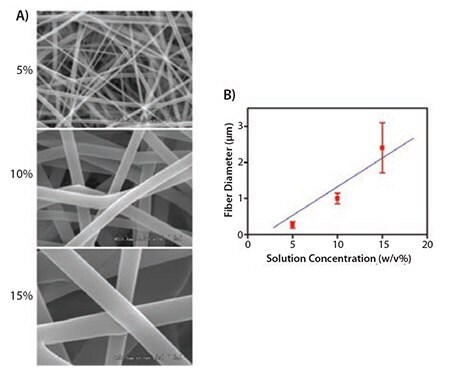
Figure 2. A) SEM images (×10K magnification) of electrospun PCL/collagen fibers with different solution concentrations. B) Average fiber diameters of electrospun PCL/collagen fibers depending on solution concentrations.
Okuda et al. demonstrated the release profiles of 5,10,15,20-tetraphenyl-21H,23H-porphine tetrasulfonic acid disulfuric acid (TPPS)-loaded or chromazural B (ChroB)-loaded electrospun fibers yielded different fiber diameters.15 For the TPPS-loaded fibers, the sustained release duration and stable quasi-linear release rate were measured as 2 h and 4.0 μg/cm2/h, respectively, for smaller fibers (160 nm), and as 4 h and 2.0 μg/cm2/h, respectively, for larger fibers (400 nm). Similarly, these parameters of ChroB-loaded fibers were measured as 1 h and 4.8 μg/cm2/h, respectively, for smaller fibers (150 nm) and as 3 h and 2.7 μg/cm2/h, respectively, for larger fibers (290 nm). Smaller fibers exhibited rapid drug release in the initial stage. Furthermore, no significant differences were observed with respect to the total amount of released drug and the fiber diameter or type of drug. These results demonstrated that the fiber diameter was inversely proportional to the drug release rate and had no measurable impact on the total quantity of drug delivered.
We previously hypothesized that the hydrophilicity of protein-carrier materials can actively influence the extent to which water diffuses through the scaffold, which is directly related to the release kinetics of the protein released from the scaffolds.16 In order to achieve controllable delivery of proteins to target locations more efficiently, we developed a novel delivery system based on electrospun fibrous poly(lactide-coglycolide) (PLGA), with a 75:25 lactide-to-glycolide ratio and molecular weight of 117 kDa, scaffolds combined with variable concentrations of PluronicR F-127 (PF-127, PEO-PPO-PEO block copolymer (Prod. No. P2443). PF-127, an amphiphilic triblock copolymer, provides hydrophilicity to the polymeric scaffolds. Hydrophilic fibrous scaffolds absorb more water; this property may facilitate diffusion of drug or protein molecules from the scaffold. In this experiment, we used BSA (Prod. No. 05470) and myoglobin (Prod. No. M5696) as model proteins to study the effects of hydrophilicity on the release of protein from electrospun nanofibrous scaffolds. The addition of PF-127, a hydrophilic polymer, to electrospun fibers significantly increased the initial burst of drug released (p ≤ 0.05) and caused a subsequent increase in the release rate. In vitro release profiles of BSA or myoglobin that were released from the electrospun PLGA nanofibers showed significant variations between fibers with different hydrophilicities (p ≤ 0.05) (Figure 3)16.
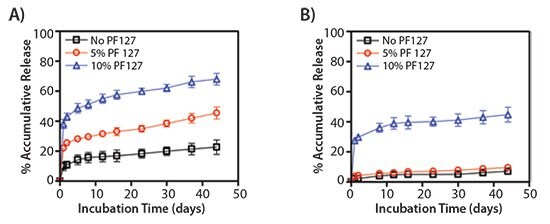
Figure 3.Release profiles of single protein delivery from the electrospun PLGA/PF-127 scaffolds. Percentage of cumulative release of A) BSA and B) myoglobin from electrospun PLGA scaffolds with different concentrations of PF-127. The release of both BSA and myoglobin could be controlled by the scaffold hydrophilicity.16
Dual Protein Delivery System Using Co-electrospinning
Previous efforts have primarily focused on the delivery of a single bioactive molecule; however, the ability to deliver multiple bioactives with distinct kinetics to drive tissue development to completion is more relevant to the clinical situation.17-21 The development of healthy tissues and organs is dependent upon the rapid remodeling of an established vasculature through the coordinated action of many bioactive molecules including growth factors, cytokines, among others.17 Moreover, sustained release of bioactive molecules with different release kinetics enables effective tissue regeneration,22 which mimics the actual in vivo tissue environment during regeneration and contributes to effective and rapid tissue regeneration.23,24
We have developed a novel dual-protein delivery system using electrospun PLGA/PF-127 fibrous scaffolds. These hydrophilic scaffolds have the potential to provide distinct sustained release profiles for multiple proteins. The hydrophilicity of the electrospun PLGA nanofibers can also be adjusted by changing PF-127 concentration during fabrication. Two model proteins, BSA and myoglobin, were incorporated successfully into the electrospun PLGA/PF-127 scaffolds and were released gradually and in a sustained manner from the scaffolds (Figure 4).16 Furthermore, the release patterns for these proteins were altered by varying the hydrophilicity of the PLGA/PF-127 composite scaffolds. This co-electrospinning technique can incorporate multiple factors by using two or more syringes and power supplies. It can be used to simultaneously electrospin two or more polymer components (Figure 5). This method has the potential to be an ideal method for the fabrication of smart biomaterial scaffolds for use in the delivery of multiple bioactive molecules in tissue engineering.
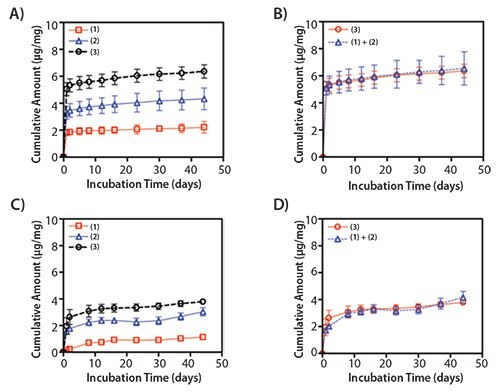
Figure 4.Release profiles of dual protein delivery from the electrospun PLGA/PF-127 scaffolds. Cumulative release amount of BSA (A,B) and myoglobin (C,D) from co-electrospun PLGA/PF-127 scaffolds. 1) PLGA-only + PLGA with 2 wt% protein (BSA or myoglobin); 2) PLGA-only + PLGA/10% PF-127 with 2 wt% protein; and 3) PLGA with 2 wt% protein + PLGA/10% PF-127 with 2 wt% protein (B,D). There was no significant difference between (1) + (2) and (3). This indicates that the co-electrospun scaffolds can deliver multiple factors with the designated release kinetics.16

Figure 5.Fluorescent images of functionalized electrospun fibers with incorporating different fluorescence dyes, DiIC (red) and DiOC (green). Co-electrospinning technique showed mixed fibrous structures with red- and green-labeled fibers simultaneously.
Three-dimensional Tissue-engineered Scaffolds
Electrospinning has evolved as a powerful tool in tissue engineering. This fabrication technology provides the ability to control properties such as biomaterial composition, fiber diameter, fiber alignment, geometry, and the degree of drug/protein incorporation in a scaffold. Nanofibers generated by electrospinning can support the adhesion and proliferation of a wide variety of cell types. More importantly, these cells maintain their phenotypic and functional characteristics on these nanofibrous scaffolds.25 Additionally, a number of recent studies have demonstrated that micro- to nano-scaled topography directly affects the adhesion, proliferation, orientation (Figure 6),26 and survival of cells in culture.27 Therefore, studies using electrospun nanofibrous scaffolds can advance our understanding of the topographical aspects of cellular interactions and guide future efforts in regenerative medicine toward improved tissue formation. Furthermore, these scaffolds can be functionalized by adding biochemical and mechanical cues such as surface modification with bioactive molecules to enhance cellular interactions for tissue engineering applications. While specific interactions between cells and functionalized electrospun scaffolds are not fully understood, knowledge gained with respect to the specific modifications that enhance cell adhesion, proliferation, and guidance of cells seeded on a scaffold, as well as those that could affect host cell infiltration, differentiation, and vascularization in vitro and in vivo will be crucial for the advancement of tissue engineering applications.

Figure 6.Immunofluorescent images of F-actin of skeletal muscle cells on electrospun PCL/collagen nanofibers: A) culture dish, B) randomly oriented, and C) aligned electrospun nanofibers (×40 magnification). Laser confocal microscopy images of F-actin of skeletal muscle cells seeded on the D) randomly oriented and E) aligned electrospun nanofibers (×600 magnification).26
Conclusions and Future Outlook
Electrospinning has evolved as an important tool in drug/protein delivery by providing the ability to control material composition, fiber diameter, and geometry in order to affect the release profile of bioactive molecules released from electrospun fibers. Bioactive molecules can be incorporated into electrospun fibers using physical adsorption, blend electrospinning, emulsion electrospinning, coaxial electrospinning as well as surface immobilization after electrospinning. Moreover, nano-scaled fiber structures generated by electrospinning can be used to improve cellular interactions, including cell adhesion, proliferation, differentiation, and ECM production allowing the control of scaffold composition, structure, and mechanical properties. The resulting electrospun nanofibrous scaffolds possess unique features and characteristics, including an extremely high surface-area-to-volume ratio that allows for enhanced cellular activities within the scaffolds. In addition, the high porosity and interconnectivity of the three-dimensional electrospun nanofibrous scaffolds provides a favorable environment for cell infiltration and attachment. The unique characteristics of electrospun nanofibers make these materials a highly promising vehicle for the future delivery of multiple bioactive factors for enhanced drug delivery and tissue engineering applications.
Acknowledgments
We would like to thank Dr. Heather Hatcher for editorial assistance. This study was supported, in part, by the Department of Energy (DE-FG02-09ER64711) and the Telemedicine and Advanced Technology Research Center (TATRC) at the U.S. Army Medical Research and Material Command (USAMRMC) through award W81XWH-07-1-0718.
References
Pour continuer à lire, veuillez vous connecter à votre compte ou en créer un.
Vous n'avez pas de compte ?